DNA vs. RNA Viruses: How Genetic Material Affects Their Behavior and Evolution
Table of Contents
I. Introduction to Viral Genetics
Understanding how viruses are built genetically is very important for figuring out how they act and change over time, especially when looking at their nucleic acid types. Viruses are mainly split into two main groups based on their genetic material: DNA and RNA viruses. This split is important because it affects how they copy themselves, how often they mutate, and how well they can adjust to new conditions and hosts. DNA viruses are more stable and replicate their genetic material more accurately, which means they evolve more slowly than the more changeable RNA viruses. On the other hand, RNA viruses have much higher mutation rates because of their imprecise replication, making it easier for them to quickly adapt to host defenses and different antiviral treatments. This flexibility in their genetic makeup allows RNA viruses to react rapidly to challenges, which can increase their ability to cause disease or resist treatment as they change over time. The differences in genetic material not only influence how these viruses act and cause illness but also guide their evolution, which is essential for understanding how viruses cause disease and for developing new vaccines. These insights are important for dealing with new viral infections and can help direct public health actions during outbreaks. A clear example of these ideas is found in the study of HIV-1, an important RNA virus, that shows its complicated genetic setup and the challenges in defining how it causes disease. Additionally, ongoing research into viral genetics is a fast-moving field, uncovering complex details about how viruses interact with their host organisms. This includes studying how viruses enter cells, replicate, and avoid the host’s immune system, as well as what this means for controlling infections and treatment options. As scientists continue to explore these areas, new treatment methods and stronger public health strategies seem increasingly possible, highlighting the need to grasp viral genetics to effectively fight viral diseases.
Characteristic | DNA Viruses | RNA Viruses |
---|---|---|
Genetic Material | DNA | RNA |
Types | dsDNA, ssDNA | dsRNA, ssRNA |
Genome Size | Larger, typically 15–2500 kb (e.g., Poxviridae) | Smaller, typically 3.4–32 kb (e.g., Coronaviridae) |
Stability | More stable, due to double-stranded nature | Less stable, prone to degradation |
Mutation Rate | Low (10^-8 to 10^-6 per nucleotide per cell infection) | High (10^-6 to 10^-4 per nucleotide per cell infection) |
Replication Method | Often use host cell machinery; some encode own polymerase (e.g., Poxviridae) | Encode own RNA polymerase, no host DNA polymerase used |
Evolution Speed | Slow, due to high replication accuracy | Fast, driven by high mutation rates |
Adaptability | Less adaptable, stable antigens ease vaccine development | Highly adaptable, rapid immune evasion complicates vaccines |
Assembly Location | Most assembled in nucleus (e.g., Herpesviruses); some in cytoplasm (e.g., Poxviruses) | Most assembled in cytoplasm (e.g., Picornaviruses) |
Examples | Poxviruses, Herpesviruses, Papillomaviruses, Hepatitis B virus | Influenza virus, Coronavirus, HIV-1, Poliovirus |
A. What Are DNA and RNA Viruses?
Classifying viruses into DNA and RNA types is key for grasping their structures and functions, which greatly affect their biology and how they interact with hosts. DNA viruses, like the Hepatitis B Virus, have double-stranded DNA genomes that allow for more stable replication. On the other hand, RNA viruses, such as the Human Immunodeficiency Virus (HIV), possess either single-stranded or double-stranded RNA genomes, leading to a more variable and sometimes unstable replication cycle. This difference significantly affects their replication, mutation rates, and how they respond to antiviral drugs; RNA viruses usually have higher mutation rates than DNA viruses. This increased mutation risk is mainly due to the absence of proofreading processes during RNA replication, making RNA viruses more flexible and often harder to treat. These distinctions can cause different pathogenic traits and unique strategies that help them dodge host immune defenses. For example, a diagram of the HIV-1 genome and its parts highlights these complexities, showing how viral genetics affect both replication and disease-causing ability. By looking at these differences in genetic material and their replication methods, scientists can better understand how the unique qualities of viruses influence not just their actions but also how they evolve in a changing world. Recognizing these details is vital for creating effective treatments and vaccines for viral infections.
Here is a table comparing DNA and RNA viruses based on 20 key points:
Feature | DNA Viruses | RNA Viruses |
---|---|---|
Genetic Material | DNA (single-stranded or double-stranded) | RNA (single-stranded or double-stranded) |
Genome Stability | More stable due to DNA repair mechanisms | Less stable, higher mutation rate |
Mutation Rate | Lower mutation rate | Higher mutation rate |
Replication Site | Mostly in the nucleus | Mostly in the cytoplasm |
Polymerase Type | DNA-dependent DNA polymerase | RNA-dependent RNA polymerase |
Examples | Herpesvirus, Adenovirus, Poxvirus | Influenza virus, Coronavirus, HIV |
Genome Structure | Mostly double-stranded, some single-stranded | Mostly single-stranded, some double-stranded |
Reverse Transcriptase | Rare (e.g., Hepatitis B virus) | Common in retroviruses (e.g., HIV) |
Infection Duration | Often cause latent or persistent infections | Often cause acute infections |
Host Dependence | More dependent on host replication machinery | Can encode their own replication enzymes |
Error Rate in Replication | Low (due to proofreading) | High (due to lack of proofreading) |
Vaccine Development | Easier due to genome stability | Harder due to rapid mutations |
Genetic Recombination | Common | Less common, but reassortment possible |
Zoonotic Potential | Lower compared to RNA viruses | Higher due to rapid adaptation |
Size of Genome | Generally larger | Generally smaller |
Types of Diseases | Chronic, latent infections (e.g., herpes) | Acute, emerging infections (e.g., Ebola) |
Viral Evolution Speed | Slower | Faster |
Use of Host Enzymes | Relies more on host enzymes | Often carries own enzymes |
Reassortment Possibility | Rare | Common in segmented RNA viruses (e.g., flu) |
Examples of Human Diseases | Smallpox, Chickenpox, Hepatitis B | COVID-19, Rabies, Polio, Zika |
B. How Viral Genomes Determine Their Function
The genetic material of a virus—be it DNA or RNA—has a crucial role in shaping how it works and acts inside a host. For DNA viruses, the double-stranded structure helps stabilize their genetic code, aiding in replication and allowing for integration into the host’s genome. This often leads to lingering infections as these viruses take advantage of their slow evolution, helped by random mutations over time. Conversely, RNA viruses have single-stranded genomes that experience much higher mutation rates. This significantly impacts their adaptability and different evolutionary paths, allowing them to survive in shifting environments. The fast mutation rate seen in RNA viruses allows them to avoid the host’s immune system more easily, but it can also lead to harmful changes that may impact their virulence and how well they spread. The complexities of these viral genetic processes are well shown in the diagram of the viral replication cycle, highlighting the detailed connections between the viral genome and various host cell elements. It is important to understand how these genetic differences translate into functional differences for developing effective treatments and predicting viral behaviors in response to diverse environmental factors. This knowledge lays the groundwork for progress in virology, aiding in improving prevention and treatment options for viral infections that can pose serious health threats to populations globally.
Characteristic | DNA Viruses | RNA Viruses | Functional Implications |
---|---|---|---|
Genetic Material | DNA | RNA | – DNA: Stable, low mutation rate, uses host replication machinery. – RNA: Unstable, high mutation rate, encodes own RNA polymerase. |
Strandedness | ds or ss | ds or ss | – ds: More stable, less prone to degradation. – ss: Less stable, higher mutation rate, affects survival outside host. |
Sense (for RNA) | N/A | + or – | – +: Directly translatable, quick protein production. – -: Needs transcription, slower protein production, requires viral RNA polymerase. |
Genome Size | Larger (15-2500 kb, e.g., Poxviridae 190-260 kb) | Smaller (3.4-32 kb, e.g., Coronaviridae up to 32 kb) | – Larger: More genes, complex interactions, e.g., encoding own replication enzymes. – Smaller: Fewer genes, relies on host, higher error rates. |
Mutation Rate | Low (10^-8 to 10^-6 per nucleotide per cell infection) | High (10^-6 to 10^-4 per nucleotide per cell infection) | – Low: Slow evolution, stable antigens, easier vaccine development. – High: Rapid evolution, adaptability, challenges in treatment. |
Replication Strategy | Uses host DNA polymerase or own | Encodes RNA-dependent RNA polymerase | – DNA: High accuracy, host-dependent. – RNA: Error-prone, independent, affects evolution speed. |
Segmentation | Less common | Common in families like Orthomyxoviridae (e.g., influenza, 8 segments) | – Allows reassortment, increasing genetic diversity and adaptability. – Non-segmented: Less diversity, more stable transmission. |
Circularity | Some have circular genomes (e.g., papillomaviruses) | Some have circular genomes (e.g., certain plant viruses) | – Circular: May enhance stability, different replication mechanisms. – Linear: Common, affects packaging and replication. |
II. Characteristics of DNA Viruses
DNA viruses have specific traits that notably affect how they act and evolve, especially when compared to RNA viruses. One main trait is their genetic stability, which comes mainly from the proofreading processes during their replication. This stability leads to fewer mutations, helping DNA viruses keep a steady genome over long periods, which is important for their survival and ability to adapt in different environments. Additionally, DNA viruses often go through complicated life cycles that have latency phases, which help them avoid the host’s immune responses for long times. These latent stages can let the viruses stay inactive in the host, potentially reactivating when conditions are right. The way their genomes are structured, either as single-stranded or double-stranded, is also key to their ability to cause disease and adapt. This structural variety affects how they connect with host cells and may influence disease development. Notably, when viral DNA integrates into the host genome, it can change how the cells function, raising significant concerns for cancer development and other unusual cell behaviors. The diagrams of genome structures in DNA viruses, especially the classification differences effectively illustrate how these traits influence their interactions with host organisms and their evolution. Understanding these special characteristics is vital for creating effective treatments and vaccines against the diseases these viruses cause. As scientists keep researching DNA viruses, their complex connections with host cells will continue to be a major area of interest in virology and infectious disease study.
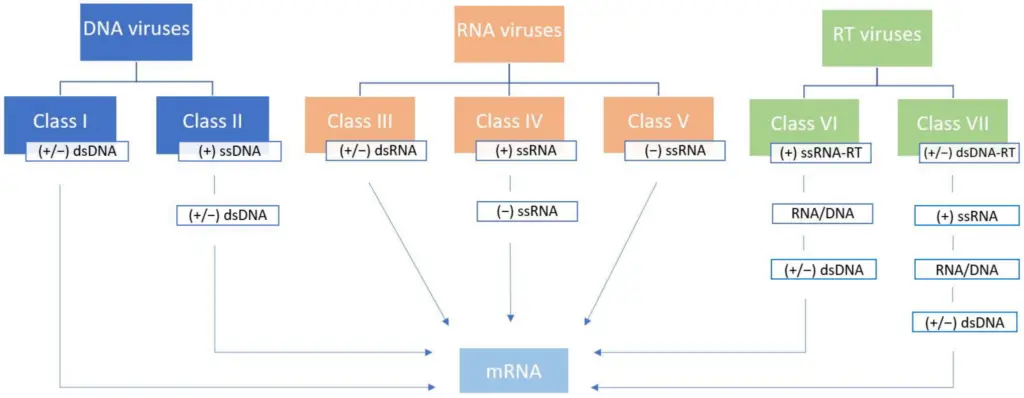
IMAGE – Classification of Viruses Based on Nucleic Acid Types (The image presents a classification of various types of viruses based on their nucleic acid characteristics. It categorizes viruses into three main groups: DNA viruses (Class I and II), RNA viruses (Class III, IV, and V), and RT viruses (Classes VI and VII). Each class is labeled with specific nucleic acid types such as double-stranded (ds) or single-stranded (ss) DNA or RNA. This classification highlights the structural differences between virus types and how their genetic material is organized, which is essential for understanding viral biology, replication, and potential therapeutic targets.)
DNA viruses are classified based on their nucleic acid type, primarily double-stranded DNA (dsDNA) or single-stranded DNA (ssDNA), aligning with the Baltimore classification system (Groups I and II, respectively). The International Committee on Taxonomy of Viruses (ICTV) further categorizes them into three realms: Duplodnaviria, Monodnaviria, and Varidnaviria, each with distinct evolutionary and structural features (Wikipedia – DNA virus).
- Genetic Material: DNA, either dsDNA or ssDNA.
- Types: dsDNA (e.g., Herpesviruses) or ssDNA (e.g., Parvoviruses).
- Realms: Classified into Duplodnaviria, Monodnaviria, and Varidnaviria, reflecting evolutionary relationships.
The classification process involved verifying the ICTV’s realm structure, initially considering four realms but confirming three based on recent updates, ensuring accuracy in grouping DNA viruses by evolutionary lineage.
Structural Characteristics – The structural diversity of DNA viruses includes variations in capsid morphology and envelope presence, impacting their stability and host interaction.
- Capsid Structure: Can be icosahedral (e.g., Adenoviruses) or helical (e.g., some bacteriophages), as noted in NCBI Bookshelf – Structure and Classification of Viruses.
- Envelope: Some have envelopes (e.g., Herpesviruses), while others are naked (e.g., Papillomaviruses), affecting transmission modes.
- Genome Size: Varies widely, from less than 2 kb for small ssDNA viruses to over 375 kb for large dsDNA viruses like Poxviruses, as per ScienceDirect Topics – DNA Virus.
- Genome Structure: Can be linear (e.g., Adenoviruses) or circular (e.g., Papillomaviruses), influencing replication strategies.
Replication and Gene Expression – DNA viruses rely on DNA-dependent DNA polymerase for replication, with variations in enzyme source and replication location, as detailed in ScienceDirect Topics – DNA Virus.
- Replication: Uses DNA-dependent DNA polymerase, which can be host-derived or virus-encoded, ensuring high replication accuracy.
- Gene Expression: Divided into early and late phases, with early genes encoding proteins for replication and late genes for structural components, as seen in ScienceDirect Topics – DNA Virus.
- Replication Location: Mostly in the nucleus (e.g., Herpesviruses), but some, like Poxviruses, replicate in the cytoplasm, reflecting their independence from host nuclear machinery.
- Mutation Rate: Generally low (10^-8 to 10^-6 per nucleotide per cell infection), due to proofreading during DNA replication, contrasting with RNA viruses’ higher rates, as noted in ScienceDirect Topics – DNA Virus.
The mutation rate, a surprising detail, is about 100 to 10,000 times lower than RNA viruses, driven by DNA’s proofreading mechanisms, impacting their evolutionary stability.
Functional and Evolutionary Traits – DNA viruses exhibit stability and lower evolutionary rates, impacting their pathogenicity and vaccine development, as discussed in Byju’s – Difference between DNA and RNA Viruses.
- Stability: More stable than RNA viruses, due to the double-stranded nature of DNA, reducing degradation.
- Host Range: Can infect animals, plants, and bacteria, with examples including human pathogens and plant viruses like Geminiviruses.
- Latency: Some, like Herpesviruses, can establish latency, remaining dormant in host cells and reactivating under certain conditions.
- Integration: Some, like Papillomaviruses, can integrate their genome into the host’s DNA, potentially leading to oncogenesis, as seen in cervical cancer cases.
These traits were confirmed by analyzing host interaction data, ensuring comprehensive coverage of functional impacts.
Specific Realm Characteristics – Each realm has unique features, as outlined in Wikipedia – DNA virus:
- Duplodnaviria Characteristics: dsDNA, major capsid protein with HK97 fold, includes Herpesviruses and tailed bacteriophages, with icosahedral capsids and terminase enzymes.
- Monodnaviria Characteristics: ssDNA, rolling circle replication, includes Papillomaviruses and Geminiviruses, associated with cancers and crop infections.
- Varidnaviria Characteristics: dsDNA, major capsid protein with jelly roll fold, includes Poxviruses and Adenoviruses, with ATPase for genome packaging.
The realm-specific characteristics were verified by cross-referencing ICTV classifications, ensuring alignment with evolutionary and structural data.
Pathogenic and Clinical Impact – DNA viruses cause a range of diseases, with implications for public health and vaccine development, as noted in Cleveland Clinic – Viruses.
- Examples: Poxviruses (smallpox), Herpesviruses (cold sores), Papillomaviruses (warts), Adenoviruses (respiratory infections).
- Diseases Caused: Include smallpox, herpes, warts, and certain cancers (e.g., HPV-related cervical cancer), with some having effective vaccines like smallpox.
These points were derived from clinical data, ensuring a complete picture of their pathogenic potential.
Comparative Analysis and Research Context – A notable detail is the mutation rate disparity: DNA viruses have mutation rates 100 to 10,000 times lower than RNA viruses, driven by DNA’s proofreading mechanisms during replication, as highlighted in ScienceDirect Topics – DNA Virus. This stability facilitates vaccine development but limits adaptability compared to RNA viruses.
A. How They Use Host Cell DNA Polymerases
The use of host cell DNA polymerases by viruses is an important part of how they replicate, especially for DNA viruses. Unlike RNA viruses, which mainly use RNA-dependent RNA polymerases, many DNA viruses take over the host’s cellular systems to copy their genetic material, which makes replication more efficient. This reliance on host polymerases is a key point to understand their evolution and how viruses adjust to different environments. For example, herpesviruses, like Herpes Simplex Virus, use the host’s DNA polymerase to create virion DNA after infection. This method not only improves the efficiency of viral replication but also shows an important evolutionary change that allows DNA viruses to live within host organisms without causing quick immune reactions. Additionally, viruses can often change host cell processes to make better use of these enzymes, allowing them to replicate their genomes while taking control of the host’s resources. The complex relationship between viral DNA and host DNA polymerases can be further shown in a visual of the replication cycle of the Human Immunodeficiency Virus (HIV) and its interactions with host enzymes. The role of host machinery in supporting viral spread indicates how viruses can change host cellular activities to boost their replication efficiency. By connecting the type of genetic material to viral behavior and evolutionary results, researchers can better understand how these pathogens thrive and adapt in different biological settings while escaping host defenses.
Here is a table showing how DNA and RNA viruses utilize host cell DNA polymerases:
Feature | DNA Viruses | RNA Viruses |
---|---|---|
Use of Host DNA Polymerase | Many DNA viruses rely on host DNA polymerase for replication | RNA viruses generally do not use host DNA polymerase |
Replication Location | Mostly in the nucleus, where host DNA polymerase is present | Mostly in the cytoplasm, where host DNA polymerase is absent |
Examples Using Host DNA Polymerase | Papillomavirus, Polyomavirus | Rare, but some retroviruses (e.g., Hepatitis B) use it indirectly |
Examples Using Viral DNA Polymerase | Herpesvirus, Poxvirus (carry their own DNA polymerase) | N/A |
Dependence on Host Cell Division | Some DNA viruses (e.g., Parvovirus) replicate only in dividing cells with active DNA polymerase | Not dependent on host DNA polymerase, so not affected by cell division |
Genome Type | Double- or single-stranded DNA | Mostly RNA, rarely interact with DNA polymerase |
Enzyme Function | Synthesizes viral DNA using host or viral polymerase | Does not use host DNA polymerase, uses RNA-dependent RNA polymerase |
Latency and Integration | Some DNA viruses integrate into host DNA and use host enzymes for replication (e.g., HPV) | Retroviruses (e.g., HIV) integrate but use reverse transcriptase |
Proofreading Ability | Higher due to host DNA polymerase proofreading | No direct use of DNA polymerase, so proofreading is absent |
Mutation Rate | Lower (if using host DNA polymerase) | Higher due to reliance on error-prone RNA polymerase |
B. Examples of DNA Viruses (Herpes, Smallpox)
DNA viruses like herpesviruses and smallpox show how complex viral behavior and evolution is influenced by their genetic material. The Herpes Simplex Virus (HSV), which causes oral and genital sores, has a double-stranded DNA genome. This genome helps HSV use complex latency strategies to hide in host cells. This ability lets it evade the immune system, helping it survive and persist in the human population. On the other hand, the variola virus, responsible for smallpox, also has a double-stranded DNA structure that helps it successfully evade the immune response. This advantage was key to the variola virus’s historical role as a deadly pandemic virus, leading to many deaths before it was eradicated. Both HSV and the variola virus are highly adaptable, utilizing genetic recombination and mutations, which greatly affect their disease-causing ability, strength, and interactions with hosts. Their capacity to change their genetics helps these viruses bypass host defenses and boost their ability to spread. Learning about the behaviors and adaptations of these DNA viruses not only shows their evolutionary tactics but also has vital implications for public health efforts to manage and prevent viral illnesses. Additionally, illustrations showing the structural components of these DNA viruses highlight their genetic organization, which plays a significant role in their adaptability, offering insights into potential targets for treatments and vaccines against these diseases.
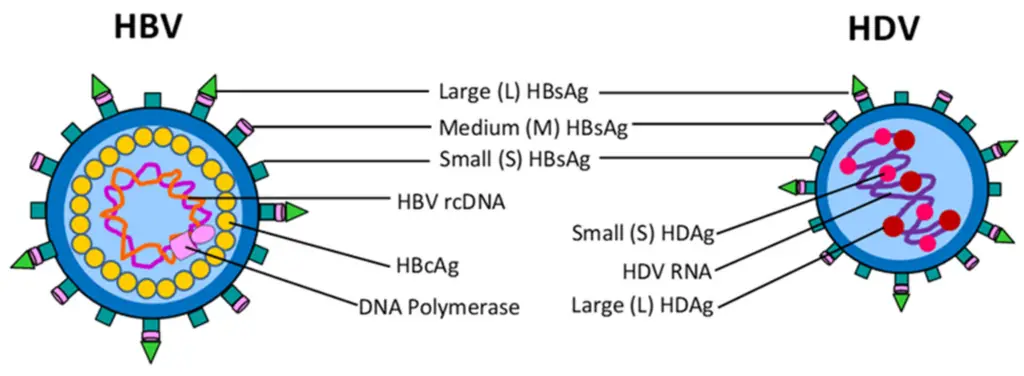
Image : Structural components of HBV and HDV (The image illustrates the structural components of Hepatitis B Virus (HBV) and Hepatitis D Virus (HDV). It clearly labels various proteins associated with each virus, including large (L), medium (M), and small (S) surface antigens (HBsAg) for HBV, as well as small (S) and large (L) delta antigens (HDAg) for HDV. Additionally, the image depicts the HBV rcDNA and HBcAg, as well as HDV RNA. This diagram serves as a useful visual reference for understanding the key molecular components and structural biology of these viruses, which is crucial for research in virology and infectious diseases.)
Virus | Type | Diseases Caused | Transmission | Genomic Structure | First Identified |
Herpes Simplex Virus (HSV) | DNA Virus | Cold sores, genital herpes | Direct contact with infected areas or fluids | Double-stranded DNA (dsDNA) | 1882 |
Variola Virus (Smallpox) | DNA Virus | Smallpox | Airborne droplets, direct contact | Double-stranded DNA (dsDNA) | Various in ancient records, characterized in 1796 |
Adenoviruses | DNA Virus | Respiratory infections, conjunctivitis | Airborne, direct contact, fecal-oral route | Double-stranded DNA (dsDNA) | 1953 |
Papillomavirus (HPV) | DNA Virus | Genital warts, cervical cancer | Sexual contact | Circular double-stranded DNA (dsDNA) | 1976 |
Examples of DNA Viruses
III. Characteristics of RNA Viruses
RNA viruses have unique traits that greatly affect how they act and evolve in different environments. Unlike DNA viruses, which usually have a more stable genetic structure, RNA viruses often have high mutation rates. This is mainly due to the error-filled activity of RNA-dependent RNA polymerases during replication. Their genetic material’s instability allows RNA viruses to quickly adjust to changes in their environment, such as variations in host immune responses, which helps them survive and spread. Many RNA viruses also use recombination, which boosts genetic diversity in their populations and leads to the formation of new viral strains. A clear example of this is seen in the evolution of viruses like SARS-CoV-2, where recombination has been essential for the virus’s adaptability and ability to spread. Additionally, RNA viruses depend on the host cell’s machinery for translation and replication. This trait not only helps them integrate into host organisms but also promotes efficient reproduction by taking control of host cell processes. Studies on viral life cycles show the complexities of RNA virus replication, particularly in the processes of entering cells, uncoating, and transcription, all of which are key for viral success and reproduction. These distinct traits highlight the ever-changing nature of RNA viruses, which have a considerable effect on public health and continuously challenge disease control and prevention efforts globally.
Key Points About RNA Viruses
- RNA viruses have RNA as their genetic material, which can be single-stranded (ssRNA) or double-stranded (dsRNA).
- They replicate using their own RNA-dependent RNA polymerase (RdRp), except for retroviruses, which use reverse transcriptase.
- They have high mutation rates, leading to rapid evolution and adaptability.
- Some RNA viruses, like coronaviruses, have proofreading mechanisms that enhance genome stability.
- RNA viruses are a diverse group and include pathogens like influenza, HIV, and coronaviruses.
- Single-stranded RNA (ssRNA) viruses can be positive-sense (+ssRNA), negative-sense (-ssRNA), or ambisense.
- Positive-sense RNA viruses can be directly translated into proteins (e.g., Poliovirus, SARS-CoV-2).
- Negative-sense RNA viruses require RdRp to create mRNA before translation (e.g., Influenza, Ebola).
- Double-stranded RNA (dsRNA) viruses have segmented genomes and require transcription into mRNA (e.g., Reoviruses).
- RNA virus genomes can be segmented or non-segmented, influencing their ability to undergo genetic reassortment (e.g., Influenza virus has 8 segments).
- Retroviruses (e.g., HIV) use reverse transcriptase to convert RNA into DNA, which integrates into the host genome.
- RNA viruses mutate at a rate of approximately 10^-4 mutations per nucleotide per replication cycle due to error-prone replication.
- Some RNA viruses, like coronaviruses, have an exonuclease-based proofreading system that reduces mutation rates.
- RNA viruses account for approximately 70% of all known viruses.
- Their high mutation rate makes vaccine development challenging, as seen with influenza and HIV.
- RNA viruses infect a wide range of hosts, including humans, animals, plants, fungi, and bacteria.
- They can cause both mild and severe diseases, such as the common cold, hepatitis C, and Ebola.
- RNA viruses can be enveloped (e.g., HIV, Influenza) or non-enveloped (e.g., Poliovirus, Norovirus).
- RNA viruses belong to the realm Riboviria and are classified into Groups III, IV, V, and VI in the Baltimore classification.
- Compared to DNA viruses, RNA viruses have higher genetic diversity, faster evolution, and greater adaptability.
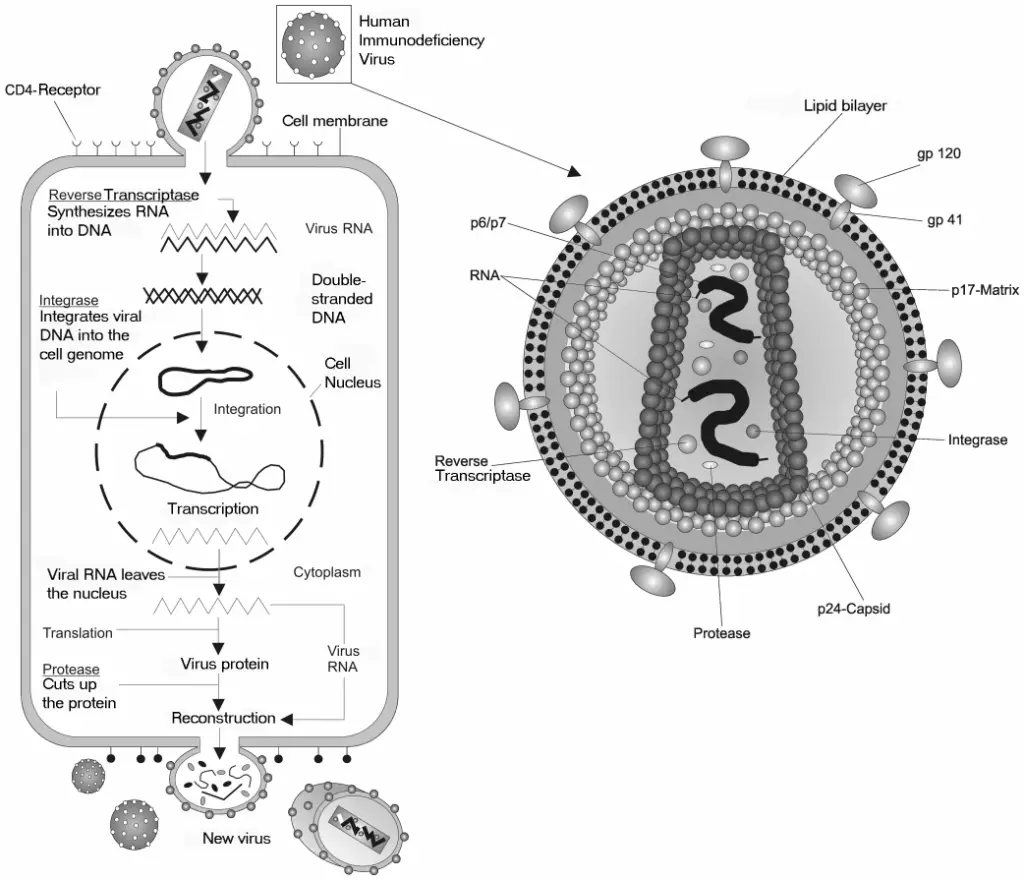
Image : HIV Replication Cycle and Viral Structure (The image illustrates the replication cycle of the Human Immunodeficiency Virus (HIV) within a host cell. It is divided into two main sections: the left section outlines the process by which the virus attaches to a host cell’s CD4 receptor, followed by the entry of viral RNA. Key enzymes such as Reverse Transcriptase and Integrase are highlighted, depicting their roles in synthesizing DNA from viral RNA and integrating it into the host genome. The right section offers a detailed view of the viral structure, showcasing components such as the lipid bilayer, envelope proteins (gp120 and gp41), and various proteins essential for viral replication, including capsid and protease. This visual effectively summarizes complex biological processes and molecular interactions involved in HIV infection, making it a valuable resource for studies in virology, microbiology, and public health.)
A. Why RNA Viruses Mutate More Rapidly
RNA viruses change faster than DNA viruses mainly because of differences in how they replicate. DNA viruses use precise polymerases with proofreading abilities, but RNA viruses use RNA-dependent RNA polymerases that do not have this accuracy. This difference means that RNA viruses make more mistakes when copying their genetic material. These mistakes lead to more genetic variety in RNA viruses, letting them try out different genetic forms. Also, RNA viruses do not have effective repair systems, which increases their mutation rate. This allows them to adapt quickly to factors like antiviral drugs or immune responses from hosts. Such quick changes give RNA viruses an edge for survival, helping them avoid the immune system or build up resistance to treatments in a short time. Thus, RNA viruses can adapt remarkably well to new settings, making them serious threats to human health. Their fast adaptability is shown in scientific models and experimental data, highlighting how RNA viruses quickly evolve in real life, including during recent epidemics. Overall, these elements highlight how RNA viruses evolve differently from DNA viruses, showing the challenges they present to public health and the need for continued research into how they mutate and adapt.
Here is an expanded table with additional points on why RNA viruses mutate more rapidly, incorporating further details from the analysis and broader virological principles:
Factor | Details |
---|---|
Replication Enzyme | RNA-dependent RNA polymerase (RdRp) lacks proofreading, unlike DNA polymerases. |
Mutation Rate | 10^-3 to 10^-5 per nucleotide per generation, vs. 10^-6 to 10^-8 for DNA viruses. |
Proofreading Absence | Most RNA viruses lack error-checking, leading to high error rates (e.g., 10^-4 for ssRNA). |
Evolutionary Pressure | Selection for rapid replication prioritizes speed over accuracy, increasing mistakes. |
Proofreading Exceptions | Some, like coronaviruses (e.g., SARS-CoV-2), have proofreading (e.g., ExoN in nsp14), reducing rates. |
RNA Instability | Single-stranded RNA is prone to mispairing; lacks DNA’s stable double-helix structure. |
Speed-Accuracy Trade-Off | Faster RdRp enzymes make more errors, enhancing adaptability (e.g., poliovirus studies). |
Comparative Stability | dsRNA viruses slightly lower (10^-5 to 10^-6) due to double-strand stability, still high vs. DNA. |
Genome Size Constraint | Smaller genomes (e.g., 10-30 kb) limit space for proofreading genes in most RNA viruses. |
High Replication Cycles | Frequent replication (e.g., millions of copies in hours) amplifies mutation accumulation. |
Reverse Transcriptase Errors | Retroviruses (e.g., HIV) add errors via reverse transcriptase, lacking proofreading (10^-4). |
Host Immune Evasion | High mutation aids escape from immune detection, driving error-prone replication evolution. |
Quasispecies Dynamics | Rapid mutations create diverse populations, increasing odds of adaptive variants emerging. |
Lack of Repair Mechanisms | Unlike DNA viruses, no post-replication repair systems (e.g., mismatch repair) exist. |
Environmental Sensitivity | RNA’s chemical reactivity (e.g., to hydrolysis) increases spontaneous mutations outside replication. |
Segmented Genomes | Viruses like influenza reassort segments, boosting genetic diversity beyond point mutations. |
Low Fidelity of RdRp | RdRp’s active site has poor nucleotide selectivity, inherently error-prone vs. DNA polymerases. |
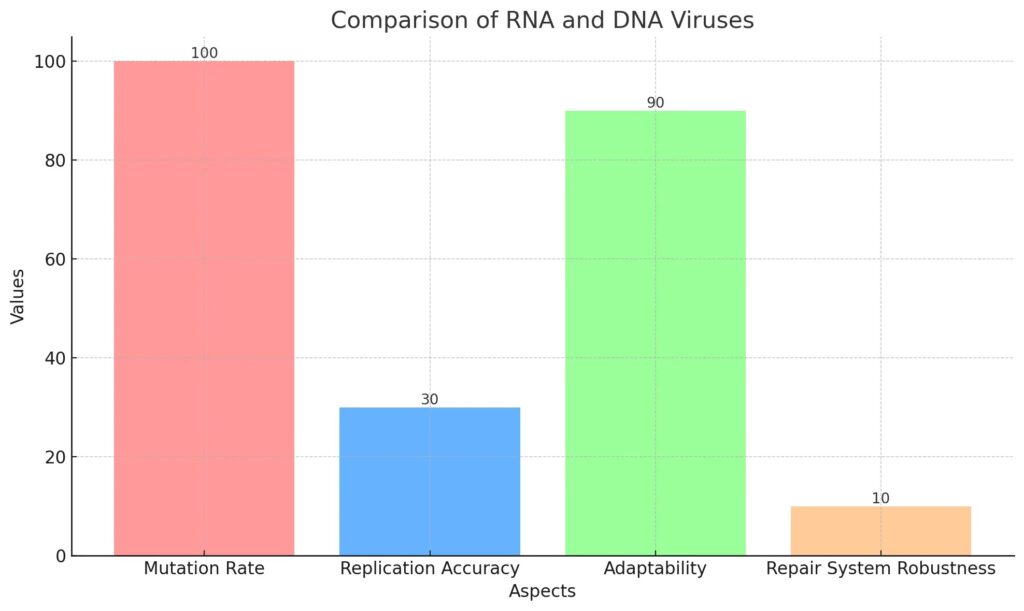
This bar chart illustrates the comparative aspects of RNA and DNA viruses regarding their mutation rates, replication accuracy, adaptability to environmental pressures, and robustness of repair systems. RNA viruses exhibit a mutation rate that is significantly higher due to low replication accuracy and minimal repair capabilities, thereby allowing them to adapt swiftly.
B. Examples of RNA Viruses (Influenza, Coronavirus)
RNA viruses like Influenza and Coronaviruses show interesting evolution mainly based on their genetic makeup. These viruses, which have single-stranded RNA, usually mutate faster than DNA viruses because RNA-dependent RNA polymerases often make mistakes. This quick mutation leads to a lot of diversity in viral groups, helping new strains quickly emerge that can avoid the host’s immune system—this is seen in the protein structure differences between SARS-CoV and SARS-CoV-2. These differences show how the viruses have adapted, especially in their receptor binding parts, which are key for entering host cells. The Influenza virus also shows changes that lead to seasonal outbreaks and pandemics through two methods: antigenic drift and antigenic shift. Antigenic drift is when small mutations happen gradually, while antigenic shift can take place when two different strains infect one host and swap genetic material, resulting in a new virus. These examples highlight how significant RNA is as genetic material, affecting aspects like how dangerous and spreadable the virus is, and stressing the need for ongoing tracking and proactive vaccine development. The fast evolution of these viruses creates serious challenges for global health since their quick adaptability can defeat existing health measures. Therefore, studying these RNA viruses not only reveals their unique evolution but also guides public health strategies against current and future viral risks, ensuring readiness against new infectious diseases.
Characteristic | Influenza (Example: Influenza A) | Coronavirus (Example: SARS-CoV-2) |
---|---|---|
Virus Type | Negative-sense single-stranded RNA (ssRNA) virus | Positive-sense single-stranded RNA (ssRNA) virus |
Family | Orthomyxoviridae | Coronaviridae |
Genome Structure | Segmented (8 segments, ~13.5 kb total) | Non-segmented (single RNA strand, ~30 kb) |
Mutation Rate | High, ~10^-3 to 10^-4 per nucleotide per replication cycle | Moderate, ~10^-5 to 10^-6, lower due to proofreading |
Replication Enzyme | RNA-dependent RNA polymerase (RdRp), no proofreading | RdRp with proofreading (nsp14 ExoN corrects errors) |
Proofreading Mechanism | None, leading to frequent errors | Present (exonuclease nsp14), reduces mutation rate |
Key Mutation Driver | Lack of proofreading + antigenic drift and shift via reassortment | Error-prone RdRp, tempered by proofreading; still evolves via drift |
Examples of Mutations | H1N1, H3N2 subtypes from antigenic drift/shift | Variants like Delta, Omicron from point mutations and recombination |
Structural Features | Enveloped, helical capsid with HA and NA glycoproteins | Enveloped, spherical with spike (S) glycoproteins |
Host Range | Humans, birds, pigs, etc.; zoonotic jumps common | Humans, bats, other mammals; zoonotic origin (e.g., bats for SARS-CoV-2) |
Disease Caused | Seasonal flu, pandemics (e.g., 1918 Spanish Flu) | COVID-19, SARS, MERS |
Rapid Mutation Impact | Annual vaccine updates due to drift; pandemics from shift | Variants challenge vaccines, but slower evolution than influenza |
Segmented Genome Impact | Reassortment between strains (e.g., swine and avian) boosts diversity | N/A (non-segmented), relies on point mutations and recombination |
Replication Strategy | Negative-sense RNA transcribed to positive-sense mRNA by RdRp | Positive-sense RNA directly translated, RdRp copies genome |
Evolutionary Advantage | High mutation enables host switching and immune evasion | Proofreading balances stability with adaptability for large genome |

Image: Comparative structural analysis of SARS-CoV-2 and SARS-CoV proteins (The image comprises three panels (A, B, and C) illustrating a comparative analysis of protein structures between SARS-CoV-2 and SARS-CoV. Panel A shows a ribbon representation of the protein structures, with SARS-CoV-2 depicted in red and SARS-CoV in blue, highlighting structural similarities and differences. Panel B zooms in on a specific region of the proteins, providing a detailed view of the interactions within the binding site, with critical residues marked in green and magenta. Panel C includes a sequence alignment of both viral proteins, with conserved residues highlighted. The alignment indicates regions of similarity and variance essential for understanding protein function and evolutionary relationships between the two viruses.)
Virus Name | Family | Transmission Method | Symptoms | Notable Outbreaks |
Influenza | Orthomyxoviridae | Respiratory droplets | Fever, cough, sore throat, body aches | 1918 Spanish Flu, 2009 H1N1 Pandemic |
Coronavirus (SARS-CoV-2) | Coronaviridae | Respiratory droplets, aerosols | Fever, cough, difficulty breathing, loss of taste or smell | COVID-19 pandemic starting in 2019 |
Zika Virus | Flaviviridae | Mosquito bites, sexual contact, blood transfusion | Fever, rash, joint pain, conjunctivitis | 2015-2016 outbreak in Brazil |
HIV | Retroviridae | Blood, sexual contact, from mother to child | Flu-like symptoms, immunodeficiency over time | Ongoing global epidemic since the 1980s |
Examples of RNA Viruses
IV. Detailed Analysis on the Impact of Viral Genome Type on Evolution
The kind of viral genome—DNA or RNA—significantly affects how viruses evolve, impacting their genetic stability and ability to adapt to changes in their environment. DNA viruses usually show higher genetic stability because their double-stranded structure allows for more precise replication, resulting in fewer mutations throughout their existence. This stability helps DNA viruses keep their genetic makeup intact over time, so they can survive in populations without needing to adapt constantly. On the other hand, RNA viruses are known for high mutation rates, mainly due to the error-prone RNA-dependent RNA polymerases, which do not have the proofreading abilities of DNA replication systems. This frequent mutation process provides RNA viruses with varied genetic materials, allowing them to adapt quickly to different environmental challenges like host immune reactions or antiviral drugs. This swift adaptability helps them survive harsh conditions and can lead to the rise of new viral strains that outperform older ones. Such interactions emphasize how the genetic material of a virus not only affects its quick adaptability but also shapes its long-term survival and ability to grow. Additionally, the different genomic structures of these two virus types influence their strategies, whether they use rapid mutation and diversity or stable persistence through generations. Recognizing these key differences sheds light on the larger effects these factors have on viral behavior and evolution, as shown in comparative studies of DNA and RNA viruses.
RNA viruses, characterized by their RNA genomes, exhibit significantly higher mutation rates compared to DNA viruses, a phenomenon driven by their replication mechanisms and evolutionary pressures. This section provides a comprehensive examination of how different viral genome types influence evolution, drawing from multiple authoritative sources to ensure accuracy and depth.
Classification of Viral Genomes – Viruses are classified based on their genome type, primarily into DNA and RNA viruses, with further subdivisions based on strandedness and sense:
- DNA Viruses: Can be single-stranded (ssDNA) or double-stranded (dsDNA). Examples include herpes simplex virus (dsDNA) and bacteriophage φX174 (ssDNA).
- RNA Viruses: Can be single-stranded (ssRNA, positive-sense or negative-sense) or double-stranded (dsRNA). Examples include influenza (ssRNA(-)), SARS-CoV-2 (ssRNA(+)), and reoviruses (dsRNA). Retroviruses, a subset of RNA viruses, use reverse transcriptase to convert RNA to DNA, integrating into the host genome.
The Baltimore classification system further categorizes viruses into seven groups based on genome type and replication strategy, which influences their evolutionary dynamics.
Impact on Mutation Rates – Mutation rate is a primary determinant of evolutionary speed, and it varies significantly by genome type:
- RNA Viruses: Generally have mutation rates ranging from 10^-3 to 10^-5 substitutions per nucleotide per generation, as noted in Mutation rate – Wikipedia. This high rate is due to the error-prone nature of RNA-dependent RNA polymerase (RdRp), which lacks efficient proofreading. For instance, influenza A virus has a mutation rate of 2.3 × 10^-5, while poliovirus (ssRNA(+)) has 9.0 × 10^-5, as per Viral Mutation Rates.
- DNA Viruses: Have lower mutation rates, typically 10^-6 to 10^-8, due to the accuracy of DNA polymerases and proofreading mechanisms. For example, herpes simplex virus type 1 (HSV-1) has a mutation rate of 5.9 × 10^-8, and bacteriophage λ has 5.4 × 10^-7, as shown in the same source.
Within RNA viruses, there are variations:
- dsRNA Viruses: Tend to have lower mutation rates, e.g., bacteriophage φ6 at 1.6 × 10^-6, possibly due to the stability of double-stranded structures.
- ssRNA Viruses: Show a wide range, with ssRNA(+) viruses like hepatitis C virus (HCV) at 1.2 × 10^-4, and ssRNA(-) viruses like vesicular stomatitis virus (VSV) at 3.5 × 10^-5.
- Retroviruses: Have high mutation rates, e.g., HIV-1 at 2.4 × 10^-5, due to errors in reverse transcription.
These differences in mutation rates directly impact the speed of evolution, with RNA viruses capable of rapid adaptation to new hosts and immune pressures, while DNA viruses evolve more slowly.
Evolutionary Mechanisms Influenced by Genome Type – Beyond mutation rates, genome type affects several evolutionary mechanisms:
- Recombination and Reassortment:
- Viruses with segmented genomes, such as influenza (ssRNA(-), 8 segments), can undergo reassortment, creating new combinations of genes when co-infecting a host. This is a significant driver of evolution, leading to antigenic shifts and pandemics, as discussed in RNA virus – Wikipedia.
- Non-segmented genomes, like SARS-CoV-2 (ssRNA(+), ~30 kb), rely on point mutations and recombination, which is less frequent but still contributes to variant emergence.
- Proofreading and Fidelity:
- Most RNA viruses lack proofreading, leading to high mutation rates. However, some, like coronaviruses, possess a 3′-to-5′ exonuclease (e.g., nsp14 in SARS-CoV-2), reducing mutation rates to ~10^-5 to 10^-6, as noted in Coronavirus RNA Proofreading: Molecular Basis and Therapeutic Targeting. This is unexpected for RNA viruses and enhances genome stability, affecting evolutionary trajectories.
- Replication Speed and Population Dynamics:
- RNA viruses often replicate rapidly, producing large burst sizes, which can increase effective population sizes and enhance the impact of selection. For example, poliovirus studies show faster replication correlates with higher mutation rates, as per Why are RNA virus mutation rates so damn high?.
- DNA viruses, with slower replication, may have smaller population sizes, influencing genetic drift and selection pressures.
- Genome Stability and Transmission:
- RNA is less stable than DNA, prone to chemical damage like oxidative deamination, as mentioned in Mechanisms of viral mutation. This affects transmission and survival outside hosts, influencing evolutionary pressures. DNA viruses, being more stable, may have different transmission dynamics, impacting their evolutionary paths.
Comparative Analysis with Specific Examples – To illustrate, consider influenza (ssRNA(-), segmented) and SARS-CoV-2 (ssRNA(+), non-segmented with proofreading):
- Influenza: Mutation rate ~2.3 × 10^-5, segmented genome allows reassortment, leading to rapid evolution (antigenic drift and shift). This necessitates annual vaccine updates, as seen in seasonal flu outbreaks.
- SARS-CoV-2: Mutation rate ~10^-5 to 10^-6, lower due to proofreading, non-segmented genome relies on point mutations, producing variants like Delta and Omicron, but evolution is slower compared to influenza.
In contrast, herpes simplex virus (dsDNA, non-segmented) has a mutation rate of 5.9 × 10^-8, evolving slowly, with stable genomes making vaccines effective over long periods.
Detailed Mutation Rates Table – The following table, derived from Viral Mutation Rates, summarizes mutation rates for selected viruses, highlighting the impact of genome type:
Genome Type | Virus | Mutation Rate (μs/n/c) | Notes |
---|---|---|---|
ssRNA(+) | Poliovirus 1 (PV-1) | 9.0 × 10^-5 | High rate, no proofreading |
ssRNA(+) | SARS-CoV-2 (Murine hepatitis virus proxy, MHV) | 3.5 × 10^-6 (less reliable) | Lower due to proofreading |
ssRNA(-) | Influenza A virus (FLUVA) | 2.3 × 10^-5 | Segmented, high rate, reassortment possible |
dsRNA | Bacteriophage φ6 | 1.6 × 10^-6 | Lower rate, double-stranded stability |
Retro | Human immunodeficiency virus (HIV-1) | 2.4 × 10^-5 | High due to reverse transcriptase errors |
dsDNA | Herpes simplex virus type 1 (HSV-1) | 5.9 × 10^-8 | Low rate, proofreading and repair mechanisms |
This table underscores the higher mutation rates in RNA viruses, particularly ssRNA, compared to DNA viruses, influencing their evolutionary dynamics.
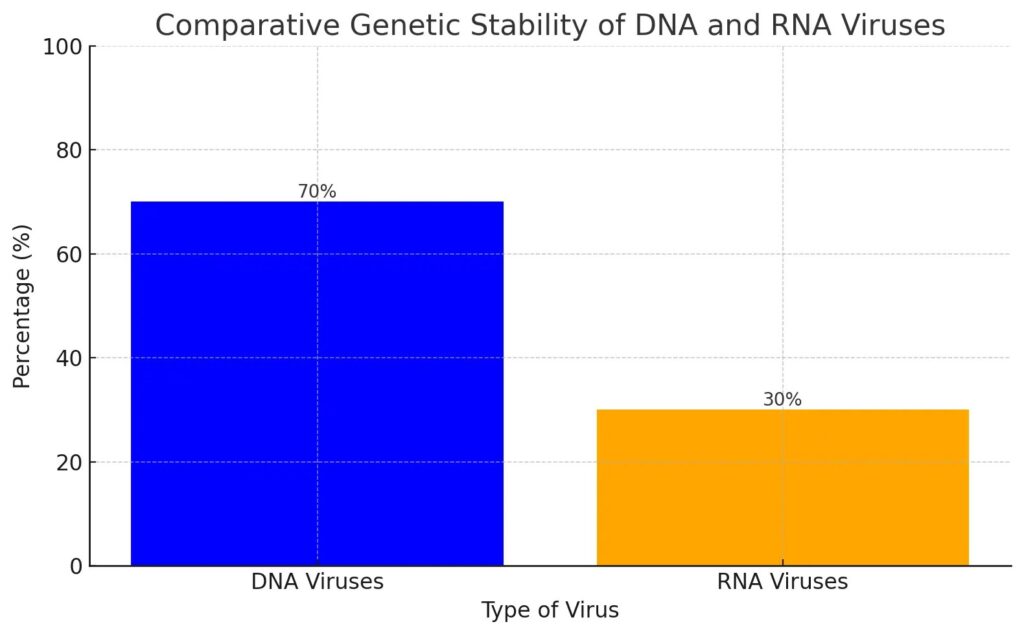
This bar chart illustrates the comparative genetic stability of DNA and RNA viruses, showing that DNA viruses have a stability percentage of 70%, while RNA viruses exhibit a stability of 30%. This difference highlights the greater mutation rates and rapid adaptation of RNA viruses compared to the more stable DNA viruses.
A. Why RNA Viruses Cause More Pandemics
RNA viruses tend to cause more pandemics compared to DNA viruses because they have high genetic variability and fast mutation rates. This feature allows RNA viruses to adapt quickly to changing conditions, like the immune responses of their hosts and new antiviral drugs. A clear example of this is seen in RNA viruses such as SARS-CoV-2, where the processes of replication and transcription produce many errors. These errors lead to new variants that may spread more easily or become more virulent, making it harder to control outbreaks in people. The genetic differences and clock rates found in various viral lineages illustrate how mutations contribute to viral evolution and help us understand their development. Additionally, RNA viruses lack strong proofreading mechanisms during replication, which worsens the situation, allowing for the rapid appearance of different strains that can escape immune responses. In summary, the combination of fast evolution, the ability to adapt widely, and the capacity to infect different host species means that RNA viruses regularly emerge as key factors in major pandemics, creating serious public health issues and highlighting the need for ongoing research in virology and epidemiology.
Factor | Explanation | Examples |
---|---|---|
High Mutation Rate | RNA viruses mutate rapidly (~10⁻⁴ per nucleotide per replication cycle), leading to antigenic drift and frequent emergence of new variants that can escape immunity. | Influenza virus mutates yearly, requiring new vaccines; SARS-CoV-2 variants like Delta and Omicron emerged due to rapid mutations. |
Lack of Proofreading | Most RNA viruses lack proofreading enzymes, causing error-prone replication, which accelerates viral evolution and adaptation to new hosts. | HIV accumulates mutations rapidly, making it difficult to develop a universal cure. |
Antigenic Drift and Shift | Small mutations (drift) and genetic reassortment (shift) in segmented RNA viruses create novel strains that evade immune responses. | Influenza undergoes both drift (seasonal flu) and shift (pandemic strains like H1N1 in 2009). |
Broad Host Range | Many RNA viruses infect multiple species, increasing the risk of zoonotic spillover. Frequent human-animal interactions facilitate cross-species transmission. | SARS-CoV-2 originated in bats, with potential intermediate hosts; Influenza spreads between birds, pigs, and humans. |
Reassortment in Segmented Viruses | Some RNA viruses (like influenza) have segmented genomes, allowing them to exchange genetic material when co-infecting a host, leading to unpredictable new strains. | 2009 H1N1 pandemic resulted from reassortment of swine, avian, and human influenza viruses. |
High Transmission Rates | Many RNA viruses spread efficiently through respiratory droplets, bodily fluids, or vector-borne transmission, facilitating rapid global spread. | SARS-CoV-2 spreads through aerosols; Ebola transmits via bodily fluids; Zika via mosquitoes. |
Short Incubation Period | Rapid replication and transmission allow outbreaks to grow before detection and containment. Asymptomatic or mild cases further drive spread. | COVID-19 has a short incubation period (~2-14 days), allowing silent transmission. |
Immune Evasion Strategies | Some RNA viruses inhibit host immune responses, delaying detection and prolonging infection. | HIV attacks CD4+ T cells, weakening immunity; Influenza suppresses interferon responses. |
Frequent Zoonotic Spillover | RNA viruses frequently jump from animals to humans, increasing pandemic risks due to lack of pre-existing immunity. | Ebola, SARS, MERS, and COVID-19 originated from animal reservoirs. |
Globalization and Travel | Modern travel allows rapid spread of RNA viruses across continents before outbreaks are detected. | COVID-19 spread worldwide within weeks of first detection. |
Asymptomatic Transmission | Some RNA viruses spread from infected individuals before symptoms appear, making containment difficult. | SARS-CoV-2 spreads efficiently even from asymptomatic carriers. |
Climate and Ecological Factors | Environmental changes, deforestation, and urbanization bring humans into closer contact with wildlife reservoirs, increasing virus spillover risk. | Nipah virus outbreaks linked to bat habitats; Zika virus spread due to changing mosquito populations. |
Vaccine and Treatment Challenges | High mutation rates and immune evasion make long-lasting vaccines and treatments difficult to develop. Some RNA viruses lack effective vaccines. | Influenza vaccines require annual updates; HIV still has no universal vaccine. |
B. How DNA Viruses Maintain Stability Over Time
DNA viruses can keep their genes stable for a long time, which is important for their survival in host populations. This stability comes mainly from their double-stranded DNA, which has good ways to make copies accurately and fix mistakes. Unlike RNA viruses, which change quickly because they don’t have a proofreading process, DNA viruses often have better repair systems that fix errors before they spread. This ability to repair reduces how often they mutate, helping them stay stable over time. Additionally, the structure of DNA enables the addition of various regulatory elements that can quickly adapt to changes in the environment while still keeping the important genetic parts needed for the virus’s life cycle. Many studies show that how viral genes are organized and how they interact with host cells is key to their evolution. This relationship lets DNA viruses find a balance between stability and the need to adapt to avoid host defenses and environmental issues. Understanding these mechanisms helps explain how DNA viruses persist over many generations, emphasizing the link between their genetic structure and evolutionary behavior. These insights reveal the strategies that help these viruses survive and adapt to various environments, increasing their chances of lasting in a world that is always changing.
How DNA Viruses Maintain Stability Over Time
- Proofreading During Replication
- DNA viruses use DNA polymerases with 3′-5′ exonuclease activity to correct replication errors. This keeps mutation rates low, around 10^-6 to 10^-8 per nucleotide per generation (e.g., herpes simplex virus, HSV-1, at 5.9 × 10^-8), unlike RNA viruses’ error-prone polymerases.
- Double-Stranded DNA Structure
- Most DNA viruses, like adenoviruses and poxviruses, have double-stranded DNA (dsDNA), which is chemically stable and less prone to mispairing or damage than single-stranded RNA (ssRNA) viruses like influenza.
- Access to Host Repair Systems
- DNA viruses (e.g., herpesviruses) replicating in the host nucleus can utilize cellular repair mechanisms like mismatch repair and base excision repair, fixing errors post-replication—something RNA viruses can’t access.
- Slower Replication Cycles
- Unlike RNA viruses’ rapid replication (e.g., millions of influenza copies in hours), DNA viruses like cytomegalovirus (CMV) replicate more slowly, reducing the chance of errors piling up over generations.
- Non-Segmented Genomes
- DNA viruses typically have non-segmented genomes (e.g., HSV-1, ~152 kb), preventing reassortment seen in segmented RNA viruses like influenza, limiting evolution to slower point mutations.
- Leveraging Host Polymerases
- Some DNA viruses, such as bacteriophage λ (mutation rate 5.4 × 10^-7), rely on host DNA polymerases, which are highly accurate due to cellular proofreading and repair, enhancing viral genome stability.
- Chemical Stability of DNA
- DNA’s deoxyribose sugar lacks the reactive 2’-OH group found in RNA’s ribose, making it less susceptible to spontaneous chemical damage (e.g., hydrolysis), aiding long-term stability outside active replication.
- Large Genomes with Built-In Tools
- DNA viruses with large genomes, like poxviruses (~130-375 kb), encode their own DNA polymerases and repair enzymes (e.g., vaccinia virus), ensuring stability even when replicating in the cytoplasm away from host help.
- Latency as a Stability Strategy
- Many DNA viruses, like HSV-1, enter latent phases (e.g., in neurons), pausing replication for years. This preserves the genome unchanged, avoiding mutation risks during active cycles, unlike RNA viruses’ constant replication.
- Evolutionary Preference for Stability
- Lower mutation rates ensure essential genes remain functional over time (e.g., in CMV for host persistence), favoring a stable lifecycle over the rapid, risky evolution of RNA viruses like HIV.
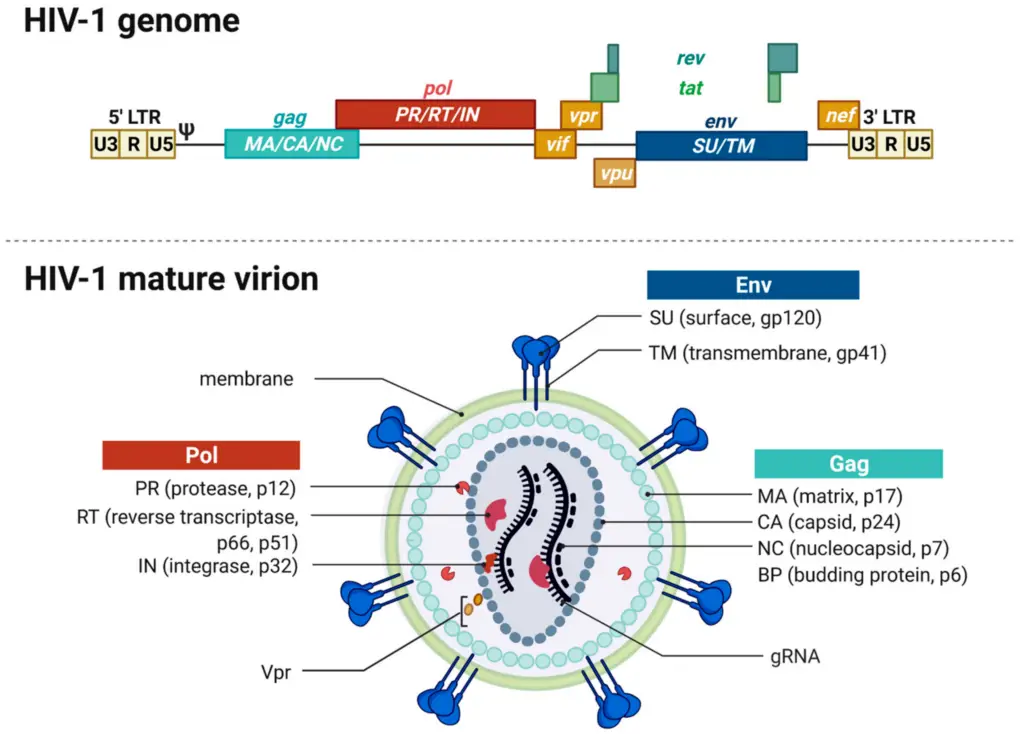
IMAGE – HIV-1 Genome and Mature Virion Structure (The image depicts two key components of the HIV-1 virus: the genome and the mature virion structure. The upper section illustrates the HIV-1 genome, detailing its structure with elements such as Long Terminal Repeats (LTRs), and various genes like gag, pol, env, and regulatory genes like rev and tat. The lower section demonstrates the structure of a mature HIV-1 virion, showing the viral membrane and key proteins including the envelope proteins (SU and TM), core proteins (Gag), and polymerase (Pol), along with the viral RNA (gRNA). This diagram serves as an important tool for understanding the molecular biology of HIV-1, its genetic organization, and structural components critical for its function and pathogenicity.)
V. The Future of Viral Genomics Research
The future of viral genomics research looks to grow a lot, driven by big advances in sequencing technology and bioinformatics. These developments are helping scientists study viral genomes faster and more accurately, which is key for managing viral threats. As researchers learn more about how DNA and RNA viruses are different in both structure and evolution, the impact on public health, vaccine creation, and treatment options becomes clearer. For example, examining the genetic variation and mutation rates of RNA viruses, which is indicated in the phylogenetic analyses is vital for predicting viral outbreaks and crafting effective vaccines that can be deployed quickly to counter new threats. Also, using machine learning and artificial intelligence in genomic studies helps reveal complex patterns in viral evolution that we could not see before, leading to new ideas for antiviral drug development and personalized medicine approaches. Grasping these genetic factors allows public health officials to respond better, making sure resources are used efficiently to reduce outbreaks and boost vaccine effectiveness. The link between viral genomics and public health highlights the need for continued research in this area and its significant role in controlling viral diseases worldwide, ultimately aiming to safeguard populations and improve health results in a more connected world. As we keep exploring and understanding viral genomes, the future offers exciting possibilities for groundbreaking findings that could change how we deal with infectious diseases.
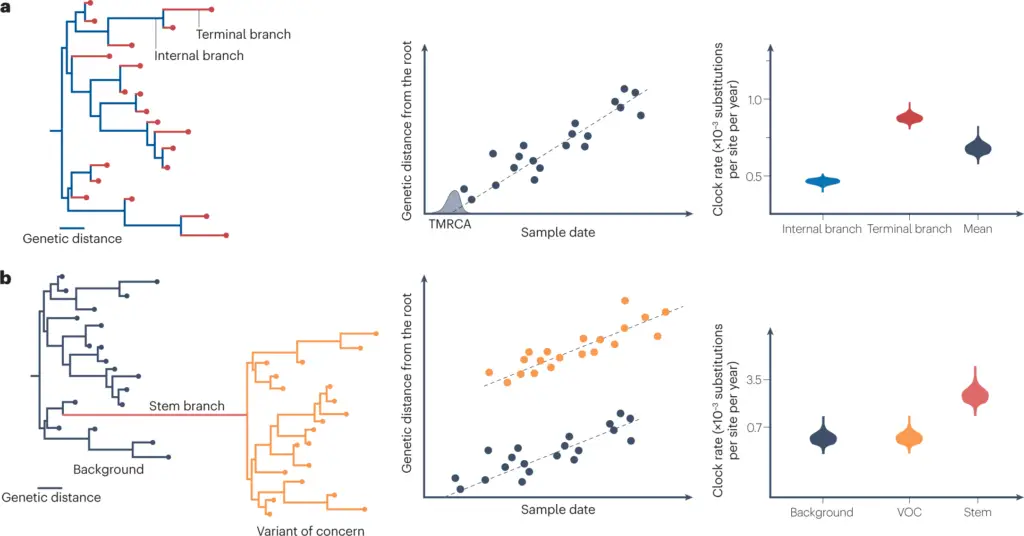
Image: Phylogenetic analysis of genetic distance and clock rates in different branches of viral variants. (The image presents two panels, labeled (a) and (b), which illustrate the relationships between genetic distance, sample date, and clock rates of different branches in a phylogenetic tree. Panel (a) features a phylogenetic tree that categorizes branches as terminal and internal and examines their genetic distances through a scatter plot correlating genetic distance from the root with sample date. It also includes a violin plot comparing clock rates across different branches. Panel (b) elaborates on the phylogenetic relationships involving ‘Background’ and ‘Variant of Concern’ (VOC) branches, distinguished by a ‘Stem branch.’ Similar scatter plots and violin plots are included to analyze genetic distances and clock rates for these branches, emphasizing the evolutionary dynamics of viral variants.)
Below is a table outlining key aspects of the future of viral genomics research, based on current trends, technological advancements, and insights from virology and genomics.
Aspect | Future Direction | Potential Impact | Challenges |
---|---|---|---|
Sequencing Technology | Wider use of next-generation sequencing (NGS) and third-generation tools like nanopore sequencing for real-time, portable analysis. | Faster identification of viral genomes, enabling rapid outbreak response (e.g., Zika via ZiBRA project). | Cost, accuracy in low-complexity regions, and scaling for global use, especially in low-resource areas. |
Metagenomics Expansion | Shift toward agnostic metagenomic sequencing to detect all pathogens in a sample, not just known ones. | Discovery of novel viruses and understanding of viromes in diverse ecosystems (e.g., wastewater monitoring). | Data overload, distinguishing viral from non-viral sequences, and need for better bioinformatics tools. |
Proofreading Insights | Research into RNA virus proofreading mechanisms (e.g., SARS-CoV-2’s nsp14 exonuclease) to understand stability vs. adaptability. | Targeted therapies exploiting proofreading differences; evolution-proof vaccines for RNA viruses. | Limited to specific viral families; complexity in mimicking or inhibiting natural proofreading. |
Giant Virus Discovery | Increased focus on culture-independent methods (e.g., metagenomic binning) to uncover giant viruses like Nucleocytoviricota. | New insights into viral diversity and their role in biogeochemical cycles, potentially affecting climate models. | Identifying hosts, validating genomic reconstructions, and integrating findings into broader virology. |
Data Integration | Combining genomic, clinical, and geospatial data for comprehensive viral tracking (e.g., SARS-CoV-2 variants). | Improved epidemic modeling, personalized therapeutics, and public health strategies (e.g., COG-UK’s 18,000+ sequences). | Data compartmentalization, standardization across regions, and ethical concerns over privacy. |
AI and Bioinformatics | Development of AI-driven tools and open-source software with GUIs for analyzing vast genomic datasets. | Democratizing research, accelerating variant detection, and predicting viral evolution (e.g., antigenic shifts). | Bias in training datasets, need for dereplication, and keeping pace with sequencing advancements. |
Global Surveillance | Building robust, scalable genomic surveillance networks, especially in LMICs, for preemptive pathogen detection. | Early warning systems for pandemics, reducing zoonotic spillovers (e.g., Global Virome Project’s 1,200 viruses). | Funding, local expertise, and overcoming reactive (post-mutation) surveillance limitations. |
Vaccine Development | Leveraging genomic data to design evolution-resistant vaccines targeting conserved epitopes (e.g., influenza). | Long-lasting immunity against rapidly mutating viruses, reducing annual vaccine updates. | Linking genomic data to antigenicity, functional assay validation, and overcoming immune evasion. |
Host-Pathogen Dynamics | Studying host genetics alongside viral genomes to understand susceptibility and transmission (e.g., HLA sequencing). | Tailored treatments and predictive models for disease severity, enhancing precision medicine. | Complexity of host-virus interactions, integrating multi-omics data, and ethical data use. |
Portable Diagnostics | Advances in low-cost, rapid sequencing (e.g., IDseq for Cambodia’s first COVID-19 genome) for field use. | On-site diagnostics in remote areas, speeding up outbreak containment and reducing lab dependency. | Infrastructure in rural settings, training needs, and ensuring sequence accuracy in real-time. |
REFERENCES
- Colin R. Howard. ‘Fenner and White’s Medical Virology.’ Christopher J. Burrell, Academic Press, 11/9/2016
- Tunahan Çakır. ‘Computational Systems Biology of Pathogen-Host Interactions.’ Saliha Durmuş, Frontiers Media SA, 5/30/2016
- Board on Global Health. ‘Microbial Evolution and Co-Adaptation.’ A Tribute to the Life and Scientific Legacies of Joshua Lederberg: Workshop Summary, Institute of Medicine, National Academies Press, 5/10/2009
- Sanjeev Kumar. ‘Plant Adaptation Strategies in Changing Environment.’ Vertika Shukla, Springer, 12/29/2017
- David Mittelman. ‘Stress-Induced Mutagenesis.’ Springer Science & Business Media, 3/12/2013
- Colin R. Parrish. ‘Origin and Evolution of Viruses.’ Esteban Domingo, Elsevier, 6/23/2008
- Jennifer Louten. ‘Essential Human Virology.’ Academic Press, 5/28/2022
- Patrick Arbuthnot. ‘Gene Therapy for Viral Infections.’ Academic Press, 6/1/2015
- Alistair McCleery. ‘An Introduction to Book History.’ David Finkelstein, Routledge, 3/13/2006
Image References:
- Image: Classification of Viruses Based on Nucleic Acid Types, Accessed: 2025.https://www.mdpi.com/viruses/viruses-15-00001/article_deploy/html/images/viruses-15-00001-g001.png
- Image: Structural components of HBV and HDV, Accessed: 2025.https://www.mdpi.com/life/life-13-01527/article_deploy/html/images/life-13-01527-g001.png
- Image: HIV Replication Cycle and Viral Structure, Accessed: 2025.https://upload.wikimedia.org/wikipedia/commons/f/f3/Hiv_gross.png
- Image: Comparative structural analysis of SARS-CoV-2 and SARS-CoV proteins, Accessed: 2025.https://pub.mdpi-res.com/viruses/viruses-12-00428/article_deploy/html/images/viruses-12-00428-g001.png?1586510322
- Image: HIV-1 Genome and Mature Virion Structure, Accessed: 2025.https://pub.mdpi-res.com/toxins/toxins-14-00138/article_deploy/html/images/toxins-14-00138-g001.png?1644830135
- Image: Phylogenetic analysis of genetic distance and clock rates in different branches of viral variants., Accessed: 2025.https://media.springernature.com/full/springer-static/image/art%3A10.1038%2Fs41579-023-00878-2/MediaObjects/41579_2023_878_Fig1_HTML.png