How Bacteria & Viruses Evolve: Mutation, Adaptation, and Resistance
Table of Contents
I. Introduction to Microbial Evolution
Microbial evolution is a dynamic and multifaceted process driven by various mechanisms, including mutation, horizontal gene transfer, and natural selection, that allows both bacteria and viruses to adapt with remarkable speed to rapidly changing environments. These microorganisms possess the unique ability to acquire new traits, often conferring significant advantages, such as antibiotic resistance, through various methods of genetic exchange. These exchange methods include transformation, where DNA is taken up from the environment; transduction, which involves the transfer of genetic material via bacteriophages; and conjugation, where genetic material is exchanged between bacteria through direct contact. For instance, the intricate interplay between genetic drift and selection pressure can lead to substantial shifts in microbial communities, ultimately influencing their overall viability and pathogenic characteristics. As illustrated in [citeX], the mechanisms that underpin genetic transfer and the development of immunity are crucial for understanding how microbes evolve and thrive amidst various environmental pressures. This ongoing evolutionary process is particularly significant given the alarming rise of antibiotic-resistant strains of microorganisms, which not only complicate treatment protocols but also pose serious threats to public health on a global scale. The emergence of these resistant strains underscores an urgent need for continuous research in the fields of microbial genetics and evolution. This research is essential in shaping effective public health strategies and therapeutic interventions aimed at combating these resilient pathogens. Understanding the genetic basis of resistance and the evolutionary pathways that lead to the dominance of certain traits over others is critical in public health planning and response strategies. Ultimately, comprehending these complex evolutionary processes is vital for effectively addressing the numerous challenges posed by increasingly resistant microbial populations, thereby safeguarding human health and enhancing our understanding of microbial dynamics in various ecosystems. Enhanced knowledge in this area not only aids in the fight against infectious diseases but also contributes to advancements in biotechnology and environmental management. Continued investment in research endeavors will foster innovative solutions to combat microbial threats, ensuring the safety and well-being of populations globally. Thus, the study of microbial evolution is not only relevant but imperative in the context of ever-evolving microbial ecosystems and their impacts on human society.
Key Aspects of Microbial Evolution – Mutation, Adaptation, and Resistance
Aspect | Definition | Mechanisms | Examples & Significance |
---|---|---|---|
Mutation | A permanent alteration in the genetic material of a microorganism. | – Spontaneous mutations due to replication errors. – Induced mutations by environmental factors (e.g., radiation, chemicals). | – Point mutations in bacteria leading to antibiotic resistance (e.g., rifampin resistance in Mycobacterium tuberculosis). – Mutations in viral genomes leading to immune evasion (e.g., antigenic drift in influenza viruses). |
Adaptation | The ability of microbes to survive and thrive in changing environments. | – Genetic recombination through horizontal gene transfer (transformation, transduction, and conjugation). – Regulatory changes enabling altered gene expression. | – Escherichia coli adapting to different nutrient sources. – Viruses evolving to evade host immune responses (e.g., SARS-CoV-2 variants). |
Resistance | The ability of bacteria and viruses to withstand antimicrobial agents. | – Acquisition of resistance genes via plasmids or transposons. – Efflux pumps removing antibiotics from bacterial cells. – Enzymatic degradation of antibiotics (e.g., beta-lactamase production). | – Multidrug-resistant Staphylococcus aureus (MRSA). – HIV developing resistance to antiretroviral therapy. – Carbapenem-resistant Enterobacteriaceae (CRE) in hospital settings. |
A. Why Bacteria & Viruses Evolve So Quickly
One of the primary reasons bacteria and viruses evolve at an accelerated rate is their unique reproductive strategies, which allow for rapid genetic variation. Bacteria, for instance, can reproduce asexually through a process known as binary fission, resulting in a near-exponential increase in population size under favorable environmental conditions. This efficient method of reproduction enables the accumulation of mutations over time, particularly when combined with horizontal gene transfer mechanisms, such as transformation and conjugation, that facilitate the sharing of genetic material among different bacterial strains. This exchange of genetic traits can significantly enhance bacterial adaptability and survival. Similarly, viruses, especially RNA viruses, often exhibit remarkably high mutation rates due to the lack of proofreading mechanisms inherent in their polymerases. This deficiency leads to diverse viral populations emerging in a remarkably short period, which can create challenges for host immune systems. Such rapid rates of mutation and subsequent adaptation are particularly critical in the context of selective pressures, including the challenges posed by antibiotic treatment or the immune responses of hosts, which drive the evolution of resistance mechanisms. Furthermore, these evolutionary trends are also influenced by environmental factors and host interactions, which can further accelerate genetic shifts. Understanding these intricate evolutionary processes is essential for developing effective strategies against bacterial and viral infections. Knowledge of how these organisms evolve can significantly inform public health responses and ongoing research efforts aimed at combating resistant strains and emerging viral threats.
Organism | Mutation Rate (per base pair per generation) | Average Generation Time (minutes) | Adaptation Examples |
Escherichia coli (E. coli) | 1.0 x 10^-10 | 20 | Antibiotic resistance genes (e.g., blaCTX-M) |
Staphylococcus aureus | 5.0 x 10^-10 | 30 | Methicillin-resistant Staphylococcus aureus (MRSA) |
Influenza virus | 1.0 x 10^-6 | 6 | Antigenic drift leading to vaccination failures |
HIV | 1.0 x 10^-5 | 2 | Drug resistance through RT mutations |
Bacteria and Viruses Evolution Statistics
II. How Bacteria Adapt and Mutate
Bacteria exhibit remarkable adaptability and mutation capabilities, allowing them to thrive in diverse and often harsh environments that would otherwise be inhospitable to many forms of life. These microorganisms utilize a range of genetic mechanisms to evolve, including mutation, gene transfer, and horizontal gene exchange, which serve as crucial tools for survival in ever-changing circumstances. Mutations can occur spontaneously during DNA replication, a natural process that can introduce random changes to their genetic material, or they can result as a direct response to environmental stressors, such as exposure to toxins or extremes in temperature and pH levels. These genetic alterations can lead to significant phenotypic changes that enhance survival rates for those bacteria facing adverse conditions. Additionally, mechanisms such as conjugation, transformation, and transduction enable bacteria to acquire resistance genes from other bacterial strains, significantly augmenting their adaptive potential and giving them a competitive edge. Conjugation involves the transfer of genetic material through direct contact, while transformation allows bacteria to uptake free DNA from their surroundings. Transduction, on the other hand, utilizes bacteriophages, or viruses that infect bacteria, to facilitate gene transfer. These processes are illustrated in the complex interactions shown in [citeX], which depicts the various methods through which antibiotic resistance can spread among bacterial populations, often at alarming rates. As bacteria encounter selective pressures, such as those stemming from antibiotic treatment or changes in their environment, they can swiftly adapt through these genetic changes, enabling them to not only survive but also to proliferate, underscoring the continual arms race between microbial evolution and medical intervention in public health contexts, which poses ongoing challenges for effective disease management and treatment strategies.
Table: Mechanisms of Bacterial Adaptation and Mutation
Mechanism | Description | Examples & Significance |
---|---|---|
Spontaneous Mutation | Random changes in DNA sequence due to errors in replication or environmental stress. | – Point mutation in Mycobacterium tuberculosis leading to rifampin resistance. – Mutation in E. coli allowing lactose metabolism in new environments. |
Induced Mutation | DNA changes caused by exposure to mutagens like UV radiation, chemicals, or reactive oxygen species. | – UV-induced mutations in bacteria leading to DNA repair system activation. – Chemical mutagens like ethidium bromide causing genetic alterations. |
Horizontal Gene Transfer (HGT) | Transfer of genetic material between bacteria, promoting rapid adaptation. | – Antibiotic resistance spread via plasmids in Klebsiella pneumoniae. – Virulence genes acquired by Vibrio cholerae through bacteriophages. |
Transformation | Uptake of free DNA from the environment into the bacterial genome. | – Streptococcus pneumoniae acquiring genes for capsule production, increasing pathogenicity. |
Transduction | Gene transfer mediated by bacteriophages (viruses that infect bacteria). | – Salmonella acquiring antibiotic resistance genes via phage-mediated transduction. |
Conjugation | Direct transfer of genetic material through pili between bacterial cells. | – Transfer of multidrug resistance plasmids in hospital-acquired infections. |
Gene Duplication & Amplification | Duplication of genes allowing functional diversification and adaptation. | – Increased expression of efflux pumps in bacteria leading to drug resistance. |
CRISPR-Cas System | Bacterial immune mechanism providing adaptive protection against viral infections. | – Escherichia coli acquiring immunity against bacteriophages. |
Phase Variation | Reversible switching of gene expression, allowing bacteria to evade host immune responses. | – Neisseria meningitidis changing surface proteins to escape immune detection. |
Quorum Sensing | Cell-to-cell communication mechanism that regulates gene expression based on population density. | – Pseudomonas aeruginosa forming biofilms in response to quorum sensing signals, enhancing antibiotic resistance. |
Bacteria Strain | Mutation Rate per Generation | Resistance Development Time in Days | Known Resistance Mechanisms |
Escherichia coli | 0.0000005 | 5-7 | Beta-lactamase, Efflux pumps |
Staphylococcus aureus | 0.0001 | 7-14 | Methicillin resistance, Biofilm formation |
Mycobacterium tuberculosis | 0.00005 | 14-30 | Multi-drug resistance, Genetic mutations |
Bacterial Adaptation and Mutation Statistics
A. Horizontal Gene Transfer and Antibiotic Resistance
Horizontal gene transfer (HGT) serves as a pivotal mechanism through which bacteria acquire antibiotic resistance, dramatically influencing their evolutionary trajectory in ways that are both profound and concerning. Unlike vertical transmission, where genetic material is passed directly from parent to offspring, HGT facilitates the exchange of genetic information between different microbial species, which can include both closely related and distantly related bacteria. This remarkable ability allows for rapid adaptation to selective pressures such as antibiotic exposure, which is particularly critical in environments where antibiotics are widely used. The processes of transformation, conjugation, and transduction exemplify how resistance genes can disseminate across bacterial populations, effectively contributing to the alarming rise of multi-drug-resistant strains that pose significant challenges to modern medicine. The prevalence of plasmids, which are mobile genetic elements that often carry multiple resistance genes, underscores the fluidity of genetic exchange in microbial communities and highlights the interconnectedness of bacterial survival strategies. This dynamic process of gene transfer enables bacteria to swiftly develop resistance to antibiotic treatments, complicating eradication efforts and threatening public health. Given the alarming increase in antibiotic-resistant infections worldwide, understanding the mechanisms and implications of HGT remains crucial for devising effective public health strategies aimed at controlling and preventing the spread of these resistant strains. This concept is further elucidated in [citeX], which visually represents HGT pathways and their implications for the escalation of antibiotic resistance, providing insight into potential intervention points that could help mitigate this critical issue.
Pathogen | Resistance Mechanism | Prevalence (%) | Year | Study Source |
Escherichia coli | Extended-Spectrum Beta-Lactamases (ESBLs) | 27 | 2022 | World Health Organization (WHO) |
Staphylococcus aureus | Methicillin-Resistant Staphylococcus Aureus (MRSA) | 42 | 2022 | Centers for Disease Control and Prevention (CDC) |
Klebsiella pneumoniae | Carbapenemase-Producing Enterobacteriaceae (CPE) | 15 | 2022 | European Centre for Disease Prevention and Control (ECDC) |
Pseudomonas aeruginosa | Multi-Drug Resistance (MDR) | 20 | 2022 | Infectious Disease Society of America (IDSA) |
Acinetobacter baumannii | Carbapenem Resistance | 30 | 2022 | National Institutes of Health (NIH) |
Horizontal Gene Transfer and Antibiotic Resistance Data
B. Bacterial Adaptations to New Environments
Bacterial adaptations to new environments are critical for their survival and success in diverse ecological niches, allowing them to thrive where other organisms may falter. One prominent mechanism that supports this adaptability is genetic exchange, which significantly enhances their ability to adapt rapidly through horizontal gene transfer. This fascinating process allows bacteria to acquire beneficial traits such as antibiotic resistance or specialized metabolic capabilities, resulting in increased survival in challenging settings. For instance, through methods like transformation, conjugation, or transduction, bacteria can become better equipped to cope with environments where nutrients may be scarce or where they face the looming threat of antimicrobial agents that could undermine their populations. The ability to form biofilms further exemplifies such adaptation; these complex communities provide a protective environment against external stressors and facilitate communal living, which optimizes resource acquisition and sharing among cells. Additionally, mutations in bacterial genomes can lead to the development of novel metabolic pathways, enabling these microorganisms to explore and exploit previously unavailable substrates for growth and energy. Such mutations can result in a competitive advantage, especially in fluctuating environments where the availability of resources may change dynamically. The schematic representation of bacterial mechanisms to resist antibiotics () vividly demonstrates these various adaptive strategies at play, underscoring the intricate interplay between genetic flexibility and environmental pressures that drives bacterial evolution. Such adaptability not only ensures the persistence of bacterial populations but also plays a significant role in shaping ecosystems as they respond to changing conditions.
Bacteria Species | Adaptation Type | Adaptation Mechanism | Environment | Study Year |
Escherichia coli | Increased antibiotic resistance | Gene mutations and plasmid acquisition | Hospital settings | 2022 |
Staphylococcus aureus | Methicillin resistance | Horizontal gene transfer | Community and hospital environments | 2023 |
Pseudomonas aeruginosa | Multidrug resistance | Biofilm formation and efflux pumps | Cystic fibrosis patients | 2021 |
Mycobacterium tuberculosis | Resistant strains emergence | Genetic mutations | High-burden TB regions | 2023 |
Vibrio cholerae | Environmental resilience | Quorum sensing and biofilm production | Brackish water ecosystems | 2022 |
Bacterial Adaptations to New Environments
III. How Viruses Evolve
Viruses exhibit remarkable evolutionary dynamics that enable them to adapt rapidly to host defenses and environmental changes, showcasing their resilience and versatility in a constantly shifting landscape. These adaptations primarily occur through various mechanisms such as mutation, genetic recombination, and reassortment, all of which allow viruses to diversify their genetic material and enhance their overall fitness. Mutations, often occurring as a result of replication errors or environmental pressures, can lead to the emergence of viral strains that manage to evade immune recognition or develop resistance to antiviral therapies that were once effective. This constant state of flux creates a scenario where the survival of the fittest principles dominate, pushing viruses to innovate continually. The process of recombination is particularly noteworthy, wherein genetic material from different viral strains is exchanged, producing novel viruses with unique properties, including increased virulence or infectivity that may pose new threats to hosts. Furthermore, the implications of these evolutionary processes are profound and far-reaching, as evidenced by the frequent emergence of new viral outbreaks, with each event underscoring the challenges posed to public health systems globally. Incorporating the concept of genetic drift and selection in viral populations further emphasizes their adaptability, as it visualizes the intricate interactions of viral and host determinants depicted in [citeX]. Overall, understanding these evolutionary strategies is essential not only for comprehending how viruses persist and thrive but also for developing effective interventions against viral diseases, enabling health professionals to stay one step ahead in the ongoing battle against these ever-evolving pathogens.
Table: Mechanisms of Viral Evolution
Mechanism | Description | Examples & Significance |
---|---|---|
Mutation | Changes in the viral genome due to errors in replication, particularly in RNA viruses that lack proofreading mechanisms. | – Antigenic drift in influenza viruses leading to seasonal flu variations. – Mutation in SARS-CoV-2 resulting in more transmissible variants (e.g., Delta, Omicron). |
Recombination | Exchange of genetic material between different viral strains when co-infecting a host cell, creating new hybrid viruses. | – Coronaviruses evolving through recombination events between bat and other animal strains. – Recombination in HIV leading to drug-resistant strains. |
Reassortment | Segmented viruses swap genome segments during co-infection, leading to major genetic shifts. | – Antigenic shift in influenza viruses causing pandemics (e.g., H1N1 in 2009). |
Natural Selection | Selective pressures favor viruses with beneficial mutations that enhance survival, transmission, or immune evasion. | – Evolution of drug-resistant HIV strains due to antiretroviral therapy pressure. – SARS-CoV-2 variants with higher infectivity outcompeting older strains. |
Genetic Drift | Random changes in viral genome frequencies over time, leading to gradual evolution. | – Minor changes in measles virus genome affecting vaccine efficacy over time. |
Host Adaptation | Viruses evolve to infect new hosts or optimize infection within a host species. | – Zoonotic transmission of Ebola and coronaviruses from animals to humans. – Adaptation of avian influenza viruses to infect mammals. |
Immune Evasion | Viruses develop mechanisms to escape host immune responses, increasing persistence. | – HIV mutating to evade neutralizing antibodies. – Herpes simplex virus (HSV) establishing latency in nerve cells. |
Latency & Persistence | Some viruses evolve strategies to remain dormant in hosts and reactivate under favorable conditions. | – Varicella-zoster virus causing chickenpox initially and reactivating as shingles. – Epstein-Barr virus (EBV) establishing lifelong infection. |
Year | Virus | Mutation Rate (nucleotide substitutions/year) | Estimated Variants Identified | Notable Variant |
2020 | SARS-CoV-2 | 1.1 | 31000 | Alpha (B.1.1.7) |
2021 | SARS-CoV-2 | 1.2 | 55000 | Delta (B.1.617.2) |
2022 | Influenza A | 2.5 | 120000 | H3N2 |
2023 | HIV | 1 | 70000 | CRF01_AE |
Viruses Evolution Statistics
A. Antigenic Drift vs. Antigenic Shift
Antigenic drift and antigenic shift are two pivotal mechanisms through which viruses, especially influenza, evolve and adapt to their environments, significantly impacting public health and vaccination strategies. Antigenic drift specifically refers to the gradual accumulation of mutations in the viral genome, which leads to small, incremental changes in the viral proteins that are recognized by the immune system. This evolutionary process allows the virus to subtly evade immune detection, making it more challenging for previously infected individuals to fight off the virus upon re-exposure. As a result, antigenic drift can contribute to the persistence of the virus within the population, often leading to seasonal outbreaks that require annual updates to vaccines. In contrast, antigenic shift represents a more dramatic change in the virus’s characteristics, usually occurring when two different strains of the virus co-infect the same host cell and subsequently exchange genetic material. This sudden genetic re-assortment can create a novel strain of the virus to which the human population has little to no pre-existing immunity. Consequently, this phenomenon has the potential to result in pandemics, as large segments of the population may be vulnerable to the new strain. Understanding these mechanisms is crucial not only for vaccine development but also for formulating effective disease prevention strategies. Such knowledge underscores the dynamic nature of viral evolution and highlights the ongoing arms race between pathogens and host immunity, emphasizing the importance of vigilance in monitoring viral changes to safeguard public health initiatives.
Table: Detailed Comparison of Antigenic Drift vs. Antigenic Shift in Viral Evolution (This table provides an in-depth comparison of antigenic drift and antigenic shift, explaining their roles in viral evolution, immune evasion, and public health implications.)
Feature | Antigenic Drift | Antigenic Shift |
---|---|---|
Definition | A gradual process of small genetic mutations in viral genes, leading to minor changes in viral surface proteins (antigens). | A sudden and major genetic change due to the reassortment of genome segments between different viral strains, leading to a novel viral subtype. |
Mechanism | – Caused by replication errors in RNA viruses, which have high mutation rates due to the absence of proofreading mechanisms. – Accumulation of point mutations (nucleotide substitutions) in genes encoding surface proteins like hemagglutinin (HA) and neuraminidase (NA). | – Occurs when two or more different strains of a segmented virus co-infect the same host cell and exchange genetic material. – Results in a completely new combination of surface antigens. |
Rate of Change | Slow, continuous, and gradual over time. | Sudden, unpredictable, and abrupt. |
Impact on Immunity & Vaccines | – Allows viruses to partially evade pre-existing immunity, leading to reduced vaccine effectiveness over time. – Requires periodic updates to vaccines (e.g., annual flu vaccine). | – Produces a virus with entirely new antigenic properties, making existing immunity ineffective. – Can lead to widespread infection due to lack of prior immune exposure. |
Host Interaction | – Results in viruses that are still recognizable by the immune system to some extent. – Can lead to seasonal epidemics due to gradual immune escape. | – Often results in a virus capable of infecting new host species or causing severe outbreaks. – Can lead to pandemics due to lack of population immunity. |
Examples in Influenza | – Seasonal influenza A and B viruses undergo antigenic drift, requiring new flu vaccines each year. – SARS-CoV-2 variants (e.g., Delta, Omicron) evolved through antigenic drift. | – Influenza A pandemics due to reassortment between human, avian, and swine flu strains: – 1918 H1N1 Spanish flu – 1957 H2N2 Asian flu – 1968 H3N2 Hong Kong flu – 2009 H1N1 swine flu pandemic |
Viral Types Involved | – RNA viruses with high mutation rates, especially influenza A, influenza B, and coronaviruses (e.g., SARS-CoV-2). – HIV also evolves through antigenic drift, leading to drug resistance. | – Only occurs in viruses with segmented genomes, such as influenza A. – Rare in other viruses but possible in segmented RNA viruses like rotavirus. |
Public Health Impact | – Requires continuous monitoring and vaccine updates. – Leads to localized outbreaks and seasonal epidemics (e.g., annual flu). | – Can cause pandemics with severe global health consequences. – Requires urgent vaccine development and global response strategies. |
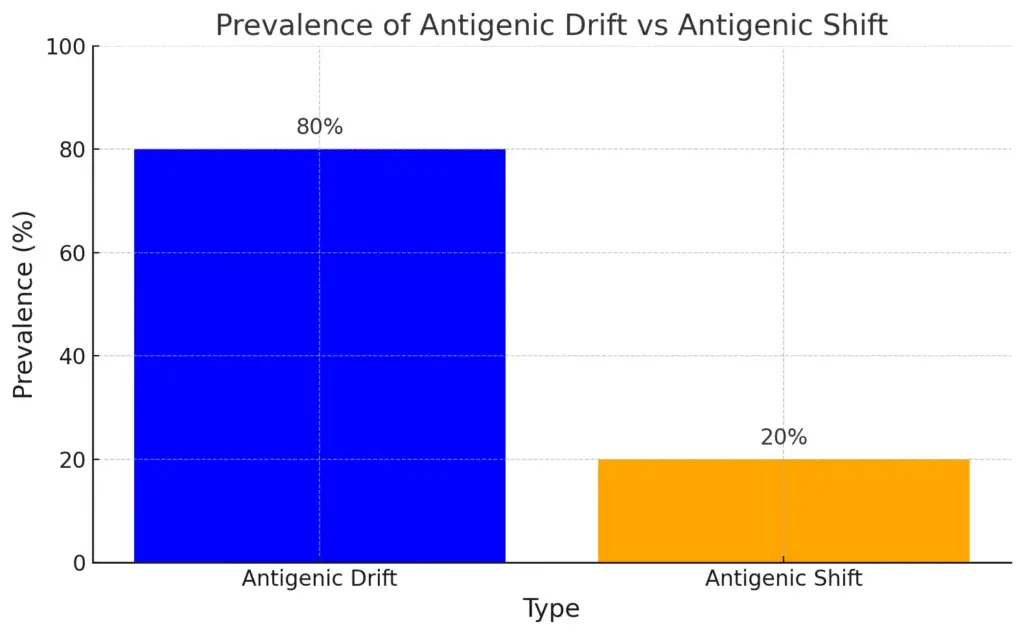
This bar chart compares the prevalence of antigenic drift and antigenic shift in viral evolution. Antigenic drift is significantly more prevalent at 80%, indicating its role in seasonal outbreaks, while antigenic shift occurs less frequently but has a major impact, accounting for 20%, and can potentially lead to pandemics.
Type | Definition | Example | Frequency | Impact | Source |
Antigenic Drift | Gradual mutations over time in virus genes that result in small changes in the antigens. | Influenza A virus seasonal variations. | Constantly occurring | Leads to yearly flu epidemics | Centers for Disease Control and Prevention |
Antigenic Shift | A major change in the virus that results from the reassortment of genes when two different strains infect a host. | Influenza A virus pandemic outbreaks. | Rare events | Can lead to pandemics with high mortality rates | World Health Organization |
Antigenic Drift vs. Antigenic Shift Data
B. Why Viruses Jump from Animals to Humans
The phenomenon of viruses jumping from animals to humans, known as zoonotic transmission, is underscored by complex evolutionary dynamics that can be traced through various contributing factors. As viruses circulate among animal populations, they undergo numerous mutations that enhance their adaptability to new hosts, which can sometimes include humans. These mutations can arise from the high replication rates of viruses, leading to a diverse genetic pool that can potentially exploit different hosts. Factors such as environmental changes, human encroachment into wildlife habitats, and increased interactions with animals further compound the likelihood of such spillover events occurring. For instance, the image of viral determinants, host determinants, and vector determinants elucidates how specific viral mutations can align with genetic vulnerabilities present in various human populations, thus facilitating transmission. Moreover, social behaviors that include the consumption of wildlife, the establishment of wet markets, and the practices surrounding animal husbandry play a significant role in facilitating contact between species, providing ample opportunities for cross-species transmission to take place. These frequent interactions not only accelerate the evolution of viruses but also pose significant public health risks, emphasizing the urgent need to enhance our understanding of viral adaptation mechanisms. With globalization and urbanization bringing humans and wildlife closer than ever, the potential for zoonotic diseases to emerge and spread is higher. Thus, raising awareness and ensuring preparedness for zoonotic diseases is critically important for mitigating future outbreaks and protecting human health, as the consequences of ignoring these dynamics can be severe and far-reaching.
Reasons Why Viruses Jump from Animals to Humans (Zoonotic Spillover)
Factor | Description | Examples & Significance |
---|---|---|
High Genetic Mutation Rates | RNA viruses, particularly coronaviruses and influenza viruses, mutate rapidly, increasing their ability to adapt to new hosts. | – SARS-CoV-2 evolved from bat coronaviruses. – Influenza viruses mutate, leading to new strains infecting humans. |
Recombination & Reassortment | Viruses with segmented genomes (e.g., influenza) can exchange genetic material between strains, enabling cross-species transmission. | – H1N1 swine flu pandemic resulted from a reassortment of bird, pig, and human flu viruses. |
Close Human-Animal Contact | Increased interactions between humans and wild or domestic animals create opportunities for viral spillover. | – Wet markets selling live animals linked to SARS and COVID-19 outbreaks. – Ebola virus linked to bushmeat hunting and consumption. |
Deforestation & Habitat Destruction | Disrupting natural ecosystems forces wildlife into closer contact with humans, increasing the risk of zoonotic transmission. | – Nipah virus outbreaks linked to bat displacement due to deforestation. – Increased human exposure to rodent-borne hantaviruses. |
Agricultural Intensification | Large-scale farming and animal husbandry create conditions where viruses can spread and evolve, increasing spillover risks. | – Avian influenza outbreaks in poultry farms. – Swine flu emerging from intensive pig farming. |
Wildlife Trade & Exotic Pet Industry | The movement of wild animals across regions facilitates the transmission of novel viruses. | – SARS outbreak traced to civet cats sold in markets. – Monkeypox cases linked to exotic pet trade. |
Climate Change & Ecological Shifts | Rising temperatures and shifting ecosystems alter animal migration and virus distribution, increasing human exposure. | – Mosquito-borne viruses like Zika and dengue expanding to new regions. – Bats migrating due to climate change, spreading coronaviruses. |
Weak Public Health Infrastructure | Poor disease surveillance and healthcare access allow zoonotic viruses to spread undetected. | – Delayed response to Ebola outbreaks in West Africa. – Limited early detection of emerging viruses in remote regions. |
Viral Adaptation & Host Jumping | Some viruses naturally evolve mechanisms to infect human cells through changes in receptor binding. | – SARS-CoV-2 acquiring mutations for better human cell entry via ACE2 receptors. – Rabies virus adapting to different mammalian hosts. |
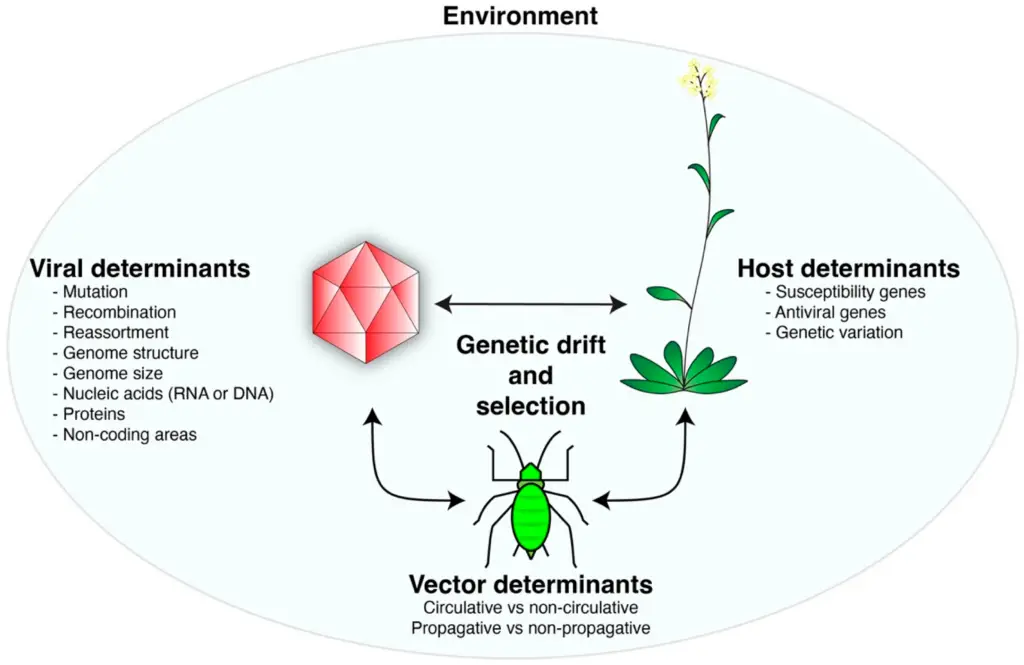
Interaction model of viral, host, and vector determinants in an environmental context (The image presents a conceptual model of interactions between viral determinants, host determinants, and vector determinants within an environmental context. It highlights various factors influencing viral behavior, including mutation, recombination, genome structure, and nucleic acids for viral determinants. Host determinants include susceptibility genes and antiviral genes, illustrating genetic variance in response to viral attacks. Additionally, vector determinants differentiate between circulative and non-circulative characteristics, as well as propagative and non-propagative traits. The concept of genetic drift and selection is emphasized as a central mechanism in mediating these interactions.)
IV. The Consequences of Microbial Evolution
The consequences of microbial evolution extend far beyond individual organisms, significantly impacting ecosystems, human health, and global public health policies, with ramifications that are increasingly noticed in today’s interconnected world. Through processes like mutation and horizontal gene transfer, bacteria and viruses can rapidly adapt to environmental changes and therapeutic pressures, leading to an increased incidence of antibiotic-resistant strains that pose a formidable challenge to modern medicine. This heightened resistance not only complicates treatment strategies but also results in longer hospital stays and higher healthcare costs, straining both patients and healthcare systems. Moreover, the evolutionary agility of microbes underscores the urgent need for surveillance and rapid development of new antimicrobial strategies to keep pace with these evolving threats. The role of pathways such as plasmid-mediated resistance illustrates how easily resistance genes can spread among bacterial populations, further exacerbating the crisis of antibiotic resistance in communities and healthcare settings alike. Such dynamics highlight the critical interplay between microbial evolution and human practices, necessitating a multifaceted approach to control and mitigate the adverse effects of these adaptations on public health outcomes. The challenge becomes even more pressing when considering the potential for these resistant strains to incorporate into the general population, affecting not only individual health but also public health at large, vividly illustrated in the mechanisms of antibiotic resistance depicted in [citeX]. As our understanding of microbial evolution deepens, it becomes increasingly clear that proactive measures, including enhanced healthcare policies, robust research initiatives, and public education, are essential in addressing the complexities introduced by evolving microbes in our world.
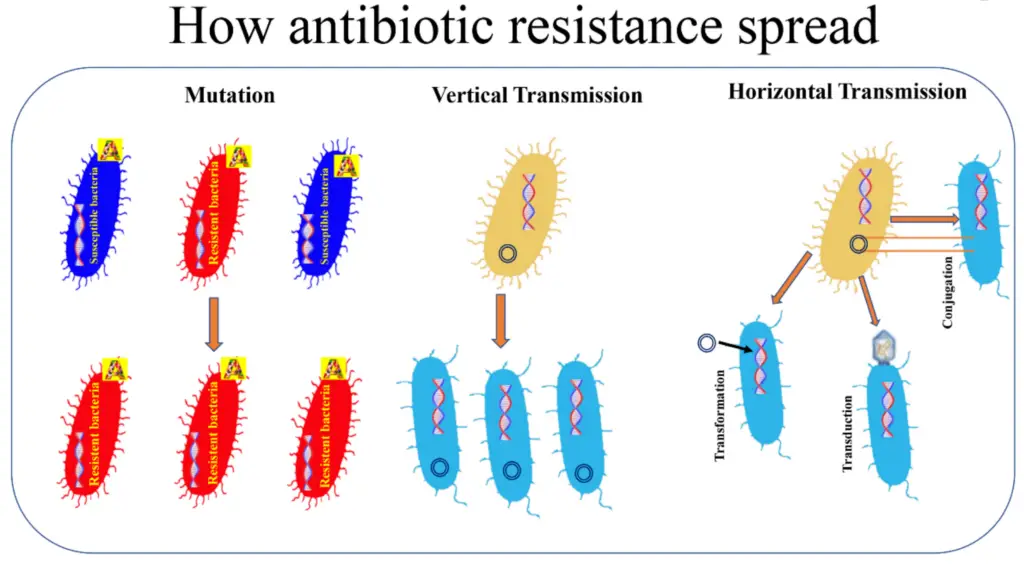
Image : Mechanisms of Antibiotic Resistance Spread (The image illustrates the mechanisms by which antibiotic resistance can spread among bacterial populations. It is divided into three sections: mutation, vertical transmission, and horizontal transmission. In the mutation section, the visual depicts both susceptible (blue) and resistant (red) bacteria, highlighting how mutations can lead to the development of resistance. The vertical transmission section demonstrates how resistant bacteria can pass their resistance traits to offspring through cellular division. The horizontal transmission section explains the processes of transformation, transduction, and conjugation, which allow bacteria to acquire resistance genes from one another, showcasing the complexity and adaptability of microbial genetics concerning antibiotic resistance.)
Consequences of Microbial Evolution
Consequence | Description | Examples & Impact |
---|---|---|
Antibiotic Resistance | Bacteria evolve mechanisms to survive antibiotic treatments, rendering medications ineffective. | – Methicillin-resistant Staphylococcus aureus (MRSA) causing difficult-to-treat infections. – Mycobacterium tuberculosis strains resistant to multiple drugs, complicating TB treatment. |
Emergence of New Infectious Diseases | Microbial evolution leads to the development of novel pathogens with pandemic potential. | – SARS-CoV-2 evolving from animal coronaviruses, causing COVID-19. – HIV emerging from simian immunodeficiency virus (SIV). |
Increased Virulence | Some pathogens evolve to become more deadly or spread more efficiently. | – Ebola virus evolving to cause more severe outbreaks. – Clostridioides difficile strains with enhanced toxin production, leading to severe diarrheal disease. |
Vaccine Escape | Viruses mutate to evade immunity from vaccines, reducing vaccine effectiveness. | – Influenza virus requiring annual vaccine updates due to antigenic drift. – SARS-CoV-2 variants reducing vaccine-induced immunity. |
Zoonotic Spillover | Pathogens adapt to infect new hosts, leading to cross-species transmission. | – Nipah virus jumping from bats to humans through contaminated food. – Avian influenza (H5N1) infecting humans from poultry. |
Biofilm Formation & Chronic Infections | Bacteria evolve to form biofilms, making infections persistent and difficult to treat. | – Pseudomonas aeruginosa forming biofilms in cystic fibrosis patients. – Dental plaque biofilms leading to cavities and gum disease. |
Immune System Evasion | Microbes develop strategies to avoid host immune responses, leading to prolonged infections. | – Mycobacterium tuberculosis surviving within immune cells. – HIV mutating to escape antibody detection. |
Microbiome Dysbiosis | Evolutionary shifts in microbial communities disrupt the balance of beneficial and harmful microbes. | – Antibiotic overuse leading to Clostridioides difficile infections. – Changes in gut microbiota linked to metabolic disorders and autoimmune diseases. |
Economic & Public Health Burdens | Increased healthcare costs, loss of productivity, and strain on medical systems. | – Billions spent annually on treating drug-resistant infections. – Global pandemics disrupting economies and healthcare infrastructures. |
A. Superbugs and Drug Resistance
The emergence of superbugs—bacteria that have developed resistance to multiple antibiotics—poses a significant challenge to global health, highlighting the critical need for new strategies to combat these formidable pathogens effectively. These superbugs evolve through various complex mechanisms such as genetic mutations, horizontal gene transfer, and the selective pressure imposed by antibiotic use, which collectively facilitate the survival of the fittest strains within microbial populations. For example, the mechanism of efflux pumps enables bacteria to actively expel harmful substances, including antibiotics, thereby enhancing their survival in hostile environments where effective treatment is crucial. Moreover, the ability of bacteria to form biofilms provides an additional layer of protection against antibiotic action, allowing these resilient organisms to thrive and proliferate despite treatment efforts that might otherwise effectively eliminate non-resistant strains. As depicted in research, understanding these intricate resistance mechanisms is not only critical for developing targeted therapies but also for fostering an environment where effective treatment protocols can be established. Ultimately, addressing the escalating threat posed by superbugs requires a multifaceted approach that combines improved antibiotic stewardship, which emphasizes the judicious use of existing antibiotics, the development of novel antimicrobial agents aimed at tackling resistant strains, and ongoing research dedicated to uncovering the dynamics of bacterial evolution and resilience. Such a comprehensive strategy is essential to safeguard public health and ensure that we can effectively combat infections in an era where superbugs are increasingly becoming a part of the microbial landscape.
Table: Superbugs and Drug Resistance – Mechanisms, Causes, and Impacts
Aspect | Description | Examples & Impact |
---|---|---|
Definition of Superbugs | Bacteria that have evolved resistance to multiple antibiotics, making infections difficult or impossible to treat. | – Methicillin-resistant Staphylococcus aureus (MRSA) causing severe skin and bloodstream infections. – Carbapenem-resistant Enterobacteriaceae (CRE), resistant to last-resort antibiotics. |
Mechanisms of Drug Resistance | Bacteria acquire genetic changes that help them survive antibiotic treatments. | 1. Enzymatic Drug Degradation – Bacteria produce enzymes that break down antibiotics (e.g., β-lactamases destroying penicillins). 2. Efflux Pumps – Bacteria actively pump antibiotics out of their cells before they can take effect. 3. Target Modification – Bacteria alter antibiotic targets so the drugs no longer bind effectively. 4. Reduced Permeability – Mutations decrease drug entry into bacterial cells. 5. Biofilm Formation – Bacteria form protective layers that shield them from antibiotics (e.g., Pseudomonas aeruginosa in cystic fibrosis lungs). |
Causes of Antibiotic Resistance | Factors contributing to the rise of drug-resistant bacteria. | – Overuse & Misuse of Antibiotics – Overprescription and misuse in humans and animals accelerate resistance. – Incomplete Antibiotic Courses – Patients stopping antibiotics too soon allow resistant bacteria to survive. – Agricultural Use of Antibiotics – Widespread antibiotic use in livestock promotes resistant bacteria. – Poor Infection Control in Healthcare Settings – Hospitals can be breeding grounds for superbugs. – Global Travel & Trade – Resistant bacteria spread across borders, increasing global health threats. |
Notable Superbugs & Their Resistance | Some of the most concerning multidrug-resistant (MDR) pathogens. | – MRSA (Methicillin-resistant Staphylococcus aureus) – Resistant to most β-lactam antibiotics, common in hospitals. – CRE (Carbapenem-resistant Enterobacteriaceae) – Resistant to nearly all antibiotics, high mortality rates. – VRE (Vancomycin-resistant Enterococci) – Causes life-threatening bloodstream infections. – MDR-TB & XDR-TB (Multidrug-resistant & Extensively drug-resistant tuberculosis) – Resistant to first-line TB drugs. – Drug-resistant Neisseria gonorrhoeae – Threatens the effectiveness of gonorrhea treatment. |
Public Health Impact | Consequences of rising antibiotic resistance on global health. | – Increased Mortality – Drug-resistant infections kill over 1.2 million people annually worldwide. – Longer Hospital Stays – Resistant infections require extended treatment, increasing healthcare costs. – Limited Treatment Options – Few effective antibiotics remain for severe infections. – Surgical & Cancer Treatment Risks – Many medical procedures rely on antibiotics to prevent infections. |
Combat Strategies & Solutions | Measures to fight antibiotic resistance and control superbugs. | – Antibiotic Stewardship – Responsible prescription and use of antibiotics. – Development of New Antibiotics – Research into novel drugs and alternative therapies. – Phage Therapy & Immunotherapy – Using bacteriophages and immune-based treatments to target resistant bacteria. – Improved Infection Control – Strengthening hospital hygiene and antimicrobial surveillance. – Public Awareness & Global Collaboration – Educating healthcare professionals and the public on responsible antibiotic use. |
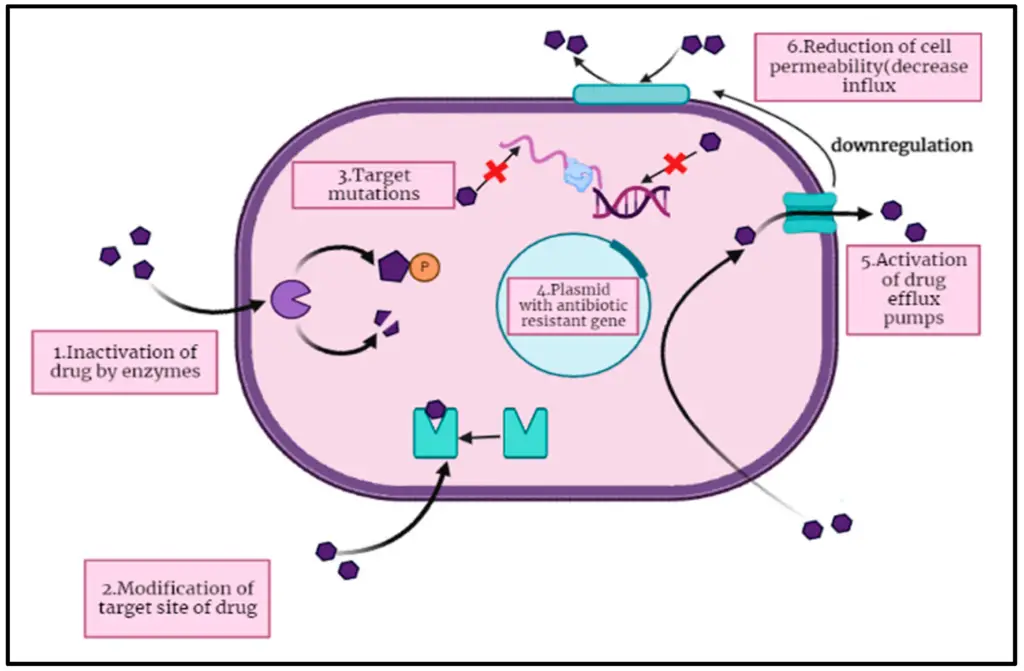
IMAGE – Mechanisms of Antibiotic Resistance in Bacteria (The image illustrates the mechanisms of antibiotic resistance in bacteria, showcasing six key processes. It depicts (1) the inactivation of the drug by enzymes, (2) modification of the drug’s target site, and (3) target mutations within the bacterial cell. It also includes (4) the presence of a plasmid containing antibiotic-resistant genes, (5) the activation of drug efflux pumps, and (6) the reduction of cell permeability to decrease drug influx. This visual representation aids in understanding how bacteria develop resistance to antimicrobial agents.)
Year | Global Deaths Due to Antimicrobial Resistance | Primary Infections |
2021 | 1.27 | Pneumonia, Tuberculosis, Sepsis |
2022 | 1.3 | Pneumonia, COVID-19, Sepsis |
2023 | 1.45 | Pneumonia, Urinary Tract Infections, Sepsis |
2023 | 3.4 | World Bank Report 2023 |
Antimicrobial Resistance Statistics
B. The Risk of Future Viral Pandemics
The increasing likelihood of future viral pandemics is underpinned by the dynamic nature of viral evolution, which is characterized by mutation, adaptation, and resistance. Viruses have the remarkable ability to rapidly modify their genetic material, often through mechanisms such as genetic reassortment and point mutations, allowing them to survive in changing environments and exploit new opportunities for infection. These evolutionary adaptations can greatly enhance their transmissibility between hosts, as observed in various influenza strains and coronaviruses that have emerged over time. Additionally, several critical factors such as global travel, rapid urbanization, and climate change significantly exacerbate the risk of spillover events, where viruses jump from animal reservoirs to humans and initiate new outbreaks. As evidenced by the COVID-19 pandemic, even relatively novel viruses can propagate swiftly and uncontrollably, highlighting the potential for widespread outbreaks that can have devastating effects on public health and global economies. The interconnectedness of our world today means that once a virus begins to spread, it can quickly cross borders, making containment efforts increasingly challenging. Proactive surveillance of zoonotic pathogens and gaining insights into viral behaviors are essential in mitigating the risks associated with these evolutionary strategies, as early detection and response can be crucial in preventing a local outbreak from escalating into a global crisis. Therefore, addressing these factors is absolutely crucial in the global effort to prepare for and combat future viral threats, encompassing not only improved scientific understanding but also international cooperation, enhanced healthcare infrastructure, and public awareness initiatives aimed at reducing the risk of future pandemics.
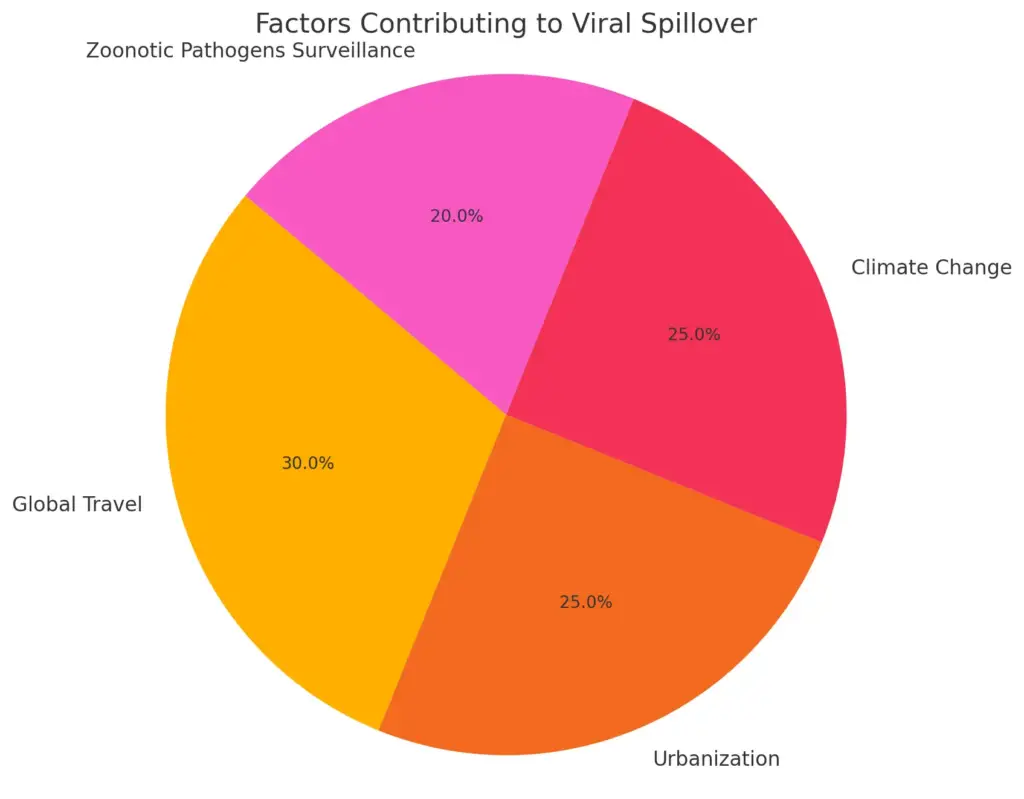
This pie chart represents the contributing factors to the risk of future viral pandemics, showing the percentage each factor contributes to the overall risk of viral spillover events. It highlights the significant role of global travel and urbanization in enhancing the likelihood of pandemics, alongside the critical need for zoonotic pathogen surveillance.
V. Conclusion – The Need for Continued Surveillance
In concluding the discussion on the evolutionary dynamics of bacteria and viruses, the imperative for continued surveillance becomes increasingly evident and pressing. With the rapid mutation rates and adaptive responses exhibited by these microorganisms, constant monitoring is essential not only to anticipate potential outbreaks but also to track the emergence of resistant strains that pose significant challenges to public health. Effective surveillance systems serve a multifaceted purpose; they not only facilitate early detection of new mutations but also provide crucial data that informs public health policies and treatment strategies designed to combat these evolving threats. Moreover, as illustrated by the interactions between viral determinants and host genetics in various studies, understanding the broader ecological context is vital for comprehending how pathogenic organisms adapt to environmental pressures that may arise due to climate change, human behavior, and other factors. The role of genetic exchange, as depicted in research findings involving horizontal gene transfer, emphasizes the importance of tracking resistance gene mobilization across different bacterial populations, which can occur rapidly and unpredictably. Thus, sustained surveillance efforts are not merely an administrative task; they serve as a proactive measure in mitigating the risks associated with microbial resistance. This ensures that health systems, policymakers, and medical professionals remain equipped to tackle evolving threats in a timely manner, allowing for rapid responses to new information, improved treatment protocols, and ultimately protecting public health on a larger scale. Continuing these efforts is crucial as we delve deeper into an era characterized by unpredictable microbial evolution.
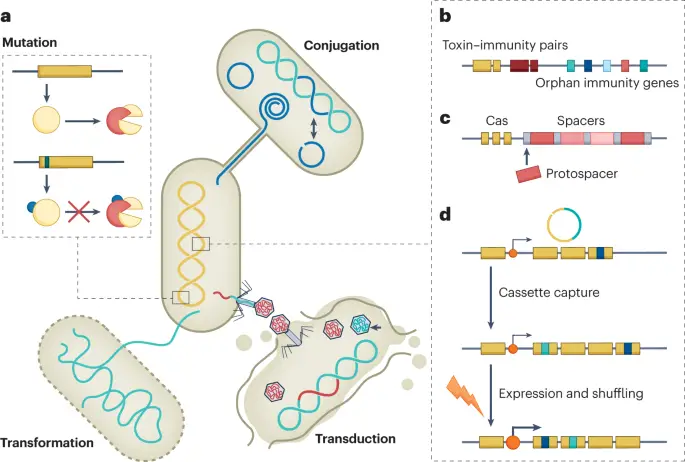
IMAGE – Mechanisms of Genetic Exchange and Immunity in Microbial Systems (The image presents a detailed diagram illustrating various genetic mechanisms involved in microbial genetic exchange and immunity. Panel (a) showcases three modes of genetic transfer: transformation, which introduces environmental DNA into a cell; conjugation, depicted as a connection between two cells for DNA transfer; and transduction, where bacteriophages introduce DNA into host cells. The inset provides a visual representation of mutations contributing to genetic diversity. Panel (b) discusses toxin-immunity pairs related to orphan immunity genes. Panel (c) delineates the relationship between CRISPR-associated (Cas) proteins and spacers in immunity context. Lastly, panel (d) describes the mechanisms of cassette capture, expression, and shuffling, highlighting genetic adaptability processes. This diagram contributes to understanding microbial genetics and the evolution of genetic traits through transfer mechanisms.)
REFERENCES
- Howard Ochman. ‘Microbial Evolution.’ Cold Spring Harbor Laboratory Press, 1/1/2016
- Board on Global Health. ‘Improving Food Safety Through a One Health Approach.’ Workshop Summary, Institute of Medicine, National Academies Press, 9/10/2012
- Jack D. Sobel. ‘Antimicrobial Drug Resistance.’ Mechanisms of Drug Resistance, Volume 1, Douglas L. Mayers, Springer, 6/19/2017
- Kunihiko Nishino. ‘Mechanisms of antibiotic resistance.’ Jun Lin, Frontiers Media SA, 6/1/2015
- Board on Global Health. ‘The Resistance Phenomenon in Microbes and Infectious Disease Vectors.’ Implications for Human Health and Strategies for Containment: Workshop Summary, Institute of Medicine, National Academies Press, 3/26/2003
- Board on Global Health. ‘Microbial Evolution and Co-Adaptation.’ A Tribute to the Life and Scientific Legacies of Joshua Lederberg: Workshop Summary, Institute of Medicine, National Academies Press, 5/10/2009
- Colin R. Parrish. ‘Origin and Evolution of Viruses.’ Esteban Domingo, Elsevier, 6/23/2008
- Isaac, Wendy-Ann. ‘Environmental Sustainability and Climate Change Adaptation Strategies.’ Ganpat, Wayne, IGI Global, 10/25/2016
- Joseph Felsenstein. ‘Inferring Phylogenies.’ Sinauer, 10/6/2003
- M.J. Waring. ‘The Genetics of Cancer.’ B.A. Ponder, Springer Science & Business Media, 12/6/2012
- Alexander Hollaender. ‘Chemical Mutagens.’ Principles and Methods for Their Detection Volume 1, Springer US, 1/4/1971
- Carlo M. Croce. ‘Holland-Frei Cancer Medicine.’ Robert C. Bast, Jr., John Wiley & Sons, 3/10/2017
- Douglas D. Richman. ‘Antiviral Drug Resistance.’ Wiley, 12/2/1996
- Eric Wajnberg. ‘Life History Evolution.’ Traits, Interactions, and Applications, Michal Segoli, John Wiley & Sons, 2/11/2025
- Alistair McCleery. ‘An Introduction to Book History.’ David Finkelstein, Routledge, 3/13/2006
Image References:
- Image: Mechanisms of Antibiotic Resistance Spread, Accessed: 2025.https://www.mdpi.com/pathogens/pathogens-10-01310/article_deploy/html/images/pathogens-10-01310-g001.png
- Image: Mechanisms of Antibiotic Resistance in Bacteria, Accessed: 2025.https://www.mdpi.com/pharmaceuticals/pharmaceuticals-16-01615/article_deploy/html/images/pharmaceuticals-16-01615-g001.png
- Image: Interaction model of viral, host, and vector determinants in an environmental context, Accessed: 2025.https://www.mdpi.com/pathogens/pathogens-11-01039/article_deploy/html/images/pathogens-11-01039-g001.png
- Image: Mechanisms of Genetic Exchange and Immunity in Microbial Systems, Accessed: 2025.https://media.springernature.com/lw685/springer-static/image/art%3A10.1038%2Fs41579-023-00877-3/MediaObjects/41579_2023_877_Fig4_HTML.png