The Electron Transport Chain: A Deep Dive into Oxidative Phosphorylation
I. Introduction
In bioenergetics, the electron transport chain (ETC) is an important part of oxidative phosphorylation, a key part of aerobic respiration. This set of protein complexes, found in the inner mitochondrial membrane, helps transfer electrons from NADH and FADH2 to molecular oxygen, which leads to ATP production, the energy source for cells. As electrons move through the chain, their energy is used to push protons across the membrane, creating a proton gradient that drives ATP synthase. This connection between electron transport and ATP production shows the ETC’s importance in cellular metabolism and energy use. Knowing how the electron transport chain works is crucial because it gives a better understanding of how cellular respiration operates and its effects on health and disease at the molecular level, opening the door for more detailed studies of its functions and importance.
A. Definition of the Electron Transport Chain (ETC)
The electron transport chain (ETC) is an important set of protein complexes and electron carriers found in the inner mitochondrial membrane and is essential for the process of oxidative phosphorylation. This series of reactions allows for the movement of electrons from NADH and FADH2, which are made during earlier parts of cellular respiration, through four main complexes (I-IV) and various mobile carriers, such as coenzyme Q and cytochrome c. Each time electrons move, protons are also pushed into the intermembrane space, creating a chemiosmotic gradient. This gradient then helps ATP synthesis happen through ATP synthase, which shows how vital the ETC is for the cell’s energy metabolism. The importance of the ETC becomes clear in situations where issues with its components can lead to mitochondrial diseases, seriously affecting cellular function and energy supply (Scheffler et al.). Therefore, learning about the ETC’s pathways is crucial for understanding both metabolic control and the importance of cellular respiration (Braeckman et al.).
B. Importance of oxidative phosphorylation in cellular respiration
The importance of oxidative phosphorylation in cellular respiration is very high, as it is the main way for ATP making in aerobic organisms. This happens in the inner mitochondrial membrane, where the electron transport chain (ETC) helps in moving electrons from donors like NADH and FADH2 through several protein complexes. As the electrons go through these complexes, protons get moved into the intermembrane space, creating a proton gradient that is key for ATP making with ATP synthase. The link between electron transport and ATP production shows how effective mitochondrial work is. If oxidative phosphorylation does not happen, this balance is upset, causing a dependence on less efficient metabolic processes, as noted in the claim that oxidative phosphorylation is the main way cells create energy as ATP, which is necessary for nearly all eukaryotic cells to live and function. This highlights the essential role of oxidative phosphorylation in keeping cellular energy balance.
C. Overview of the essay structure
In making a detailed look at the electron transport chain (ETC) as part of oxidative phosphorylation, the essay uses a clear layout to explain tough biochemical ideas. Starting with what mitochondria do, the essay shows why the ETC is important in cellular respiration, focusing on how it helps make ATP. Following sections outline the different protein complexes involved, where the movement of electrons from sources like NADH and FADH2 is closely looked at to explain how protons are pumped and how electrochemical gradients work. The discussion then moves to what happens when mitochondria do not work properly, pointing out links to various diseases and stressing the connection between energy failure and medical conditions ((Kuang et al.)). In the end, the conclusion brings together insights, repeating the vital role of the electron transport chain in keeping energy balance in cells and its possible use in future mitochondrial treatments.
II. The Structure and Components of the Electron Transport Chain
The structure and parts of the electron transport chain (ETC) are important in oxidative phosphorylation, which is a main way to produce ATP. The ETC has four protein complexes (I-IV) that are in the inner mitochondrial membrane. These complexes work in order to move electrons from NADH and FADH2 to oxygen, making a proton gradient across the membrane. This gradient helps make ATP through ATP synthase. New research shows that these protein complexes are not fixed; they are changing. There are different ideas, like solid, fluid, and plasticity frameworks, to describe how they are arranged and how they interact ((Díaz Moreno et al.)). Also, cytochrome c is a key link that improves the efficiency of electron transfer between complexes III and IV and reduces the making of reactive oxygen species, which is essential for cellular energy production ((Scheffler et al.)).
A. Description of the mitochondrial inner membrane
The mitochondrial inner membrane is important for cellular respiration because it holds the electron transport chain (ETC) and ATP synthase, creating the necessary space for oxidative phosphorylation. Its high surface area, due to folds known as cristae, helps in making the proton gradient needed for ATP production. The way protein complexes are arranged in the inner membrane is still being studied, with current theories, like the plasticity model, showing how their setup affects electron flow and proton movement efficiency (Díaz Moreno et al.). Moreover, the membrane’s inability to allow protons to pass through underscores its role in keeping the proton motive force, which is necessary for making ATP (Biousse et al.). Since the structure of the inner membrane is vital, any changes—like mutations that impact OPA1—can mess up mitochondrial function, suggesting that its structural soundness is essential for ensuring energy balance and function in eukaryotic cells.
B. Key protein complexes involved in the ETC
The electron transport chain (ETC) has four main protein complexes: Complex I, II, III, and IV, plus mobile electron carriers like coenzyme Q and cytochrome c. Each part is important for moving electrons from NADH and FADH2 to oxygen, which helps pump protons across the inner mitochondrial membrane, creating a proton gradient needed for ATP production. New studies show that stable protein structures in the ETC are important; disruptions can harm mitochondrial function and lead to different diseases. It is noted that the proteins in this chain tend to stay stable in mitochondria when membrane structure has been compromised, showing how these complexes are tough under stress.
“The proteins making up this chain are likely to remain relatively stable in mitochondria whose membrane integrity has been lost, which allows measurements that indicate the maximum capacity of the mitochondria to produce ATP to be taken.”
The complex interactions among these parts not only improve electron flow but also reduce the creation of reactive oxygen species, thus boosting the efficiency of oxidative phosphorylation.
Complex | Function | Proton Pumps | Number of Subunits | Energy Yield (ATP) |
Complex I (NADH:ubiquinone oxidoreductase) | Oxidizes NADH and transfers electrons to ubiquinone | Yes | 45 | 2.5 |
Complex II (Succinate:ubiquinone oxidoreductase) | Oxidizes succinate and transfers electrons to ubiquinone | No | 4 | 1.5 |
Complex III (Ubiquinol:cytochrome c oxidoreductase) | Transfers electrons from ubiquinol to cytochrome c | Yes | 11 | 1.5 |
Complex IV (Cytochrome c oxidase) | Transfers electrons from cytochrome c to molecular oxygen | Yes | 13 | 1 |
Key Protein Complexes in the Electron Transport Chain
C. Role of electron carriers in the transport process
Electron carriers are very important in the electron transport process. They help move electrons through the mitochondrial inner membrane during oxidative phosphorylation. Key molecules like NADH and FADH2 donate electrons to the chain, starting a series of redox reactions that are vital for energy production. These carriers act as substrates for the electron transport chain and also help keep electrochemical gradients by pumping protons (H+) across the membrane. This pumping supports the proton motive force needed for ATP synthesis. New studies show that cytochrome c acts as a soluble electron carrier and, with its additional binding spots on complexes III and IV, creates a limited diffusion path that boosts electron transfer efficiency while reducing reactive oxygen species production (Díaz Moreno et al.). So, the coordination of electron carriers is crucial for improving cellular respiration processes and energy output.
III. Mechanism of Electron Transfer
The mechanism of electron transfer within the electron transport chain (ETC) is integral to the process of oxidative phosphorylation, highlighting the dynamic interplay between various protein complexes embedded in the inner mitochondrial membrane. As electrons derived from NADH and FADH2 traverse through complexes I-IV, they drive protons (H+) from the mitochondrial matrix into the intermembrane space, generating a proton gradient that is fundamental for ATP synthesis via ATP synthase. Each complex not only facilitates electron transfer but also performs critical redox reactions, ultimately reducing molecular oxygen to form water—a crucial end product of aerobic respiration. Complex I, for example, is often implicated in mitochondrial dysfunction due to its multifactorial interactions and the discovery of numerous mutations associated with complex I deficiencies. This elucidation of electron transfer reinforces the substantial role of mitochondrial efficiency in broader metabolic processes, pivotal for cellular energy homeostasis and overall organismal health.
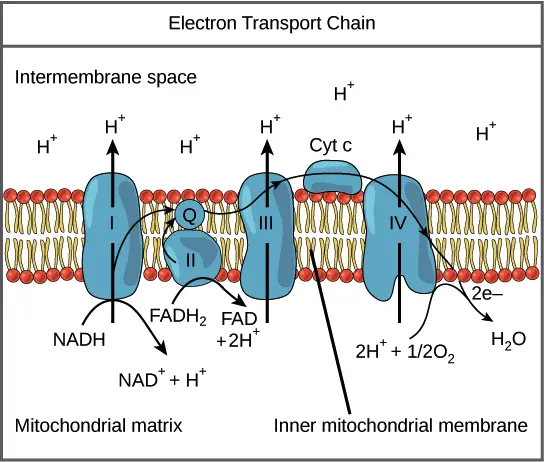
Image1 : Diagram of the Electron Transport Chain in Cellular Respiration
A. The process of electron donation and acceptance
In the electron transport chain (ETC), the donation and acceptance of electrons is key to oxidative phosphorylation. First, electrons from NADH and FADH2 go to four protein complexes in the inner mitochondrial membrane, starting a set of redox reactions. As electrons pass through the complexes, they do two main things: they move protons across the membrane and help create a proton gradient. The build-up of protons in the intermembrane space results in a chemiosmotic potential, which ATP synthase uses to make ATP from ADP and inorganic phosphate when protons flow back into the mitochondrial matrix. In the end, electrons are accepted by molecular oxygen, reducing it to water. This shows how dependent electron transfer, energy conversion, and metabolic efficiency are in cellular respiration (Abudhaise et al.), (Ramsaroop et al.).
B. The role of redox reactions in energy conversion
Redox reactions are vital for energy changing, especially in the electron transport chain (ETC) during oxidative phosphorylation. These reactions involve moving electrons, which helps create a proton gradient across the mitochondrial membrane and supports making adenosine triphosphate (ATP). The flow of electrons from Complexes I to IV shows a chain of oxidation-reduction reactions that result in turning molecular oxygen into water, an important finish for aerobic respiration. In this process, mitogen-activated protein (MAP) kinases help link redox signaling with controlling metabolism, which improves how cells adjust to energy needs. As noted, catalysts are key in improving the efficiency of both OER and HER by reducing the activation energy for these reactions.
“Catalysts play an important role in enhancing the efficiency of both OER and HER by lowering the activation energy required for these reactions. Platinum remains the benchmark catalyst for HER due to its exceptional electrocatalytic activity, while cobalt- and nickel-based materials are commonly used for OER, offering a cost-effective alternative.”
Such processes highlight the complex connections between redox reactions and managing energy in cells, showing their significant role in biochemistry.
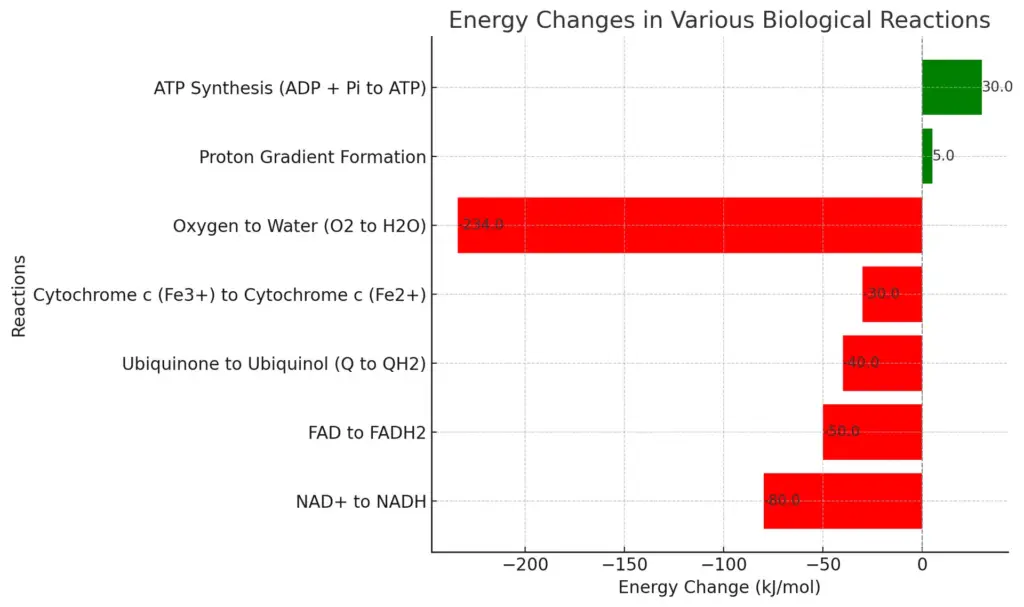
The chart displays the energy changes associated with various biological reactions. Negative values indicate energy-releasing reactions (reductions), while positive values denote energy-consuming processes. The most significant reduction occurs in the reaction converting Oxygen to Water, reflecting a highly exergonic process. Conversely, ATP synthesis is the primary energy-consuming reaction shown. The chart clearly differentiates between the types of reactions through color coding and provides a straightforward visualization of energy changes in kilojoules per mole.
C. The significance of the proton gradient in ATP synthesis
The proton gradient across the inner mitochondrial membrane is an important part of how cells create energy, helping in the making of ATP through oxidative phosphorylation. This gradient forms during electron transport, when protons are pumped from the mitochondrial matrix into the space between the membranes, creating a difference in concentration that serves as a source of potential energy. As mentioned, the gradient produced by the electron transport chain drives ATP creation through oxidative phosphorylation, which is vital for the energy needs of the cell (Shah et al.). This gradient not only allows protons to flow back into the matrix through ATP synthase but also connects the energy gained from electron transport to the conversion of ADP into ATP. In the end, the importance of the proton gradient is central to energy metabolism in cells, highlighting its key role in supporting physiological functions and maintaining balance within the cell.
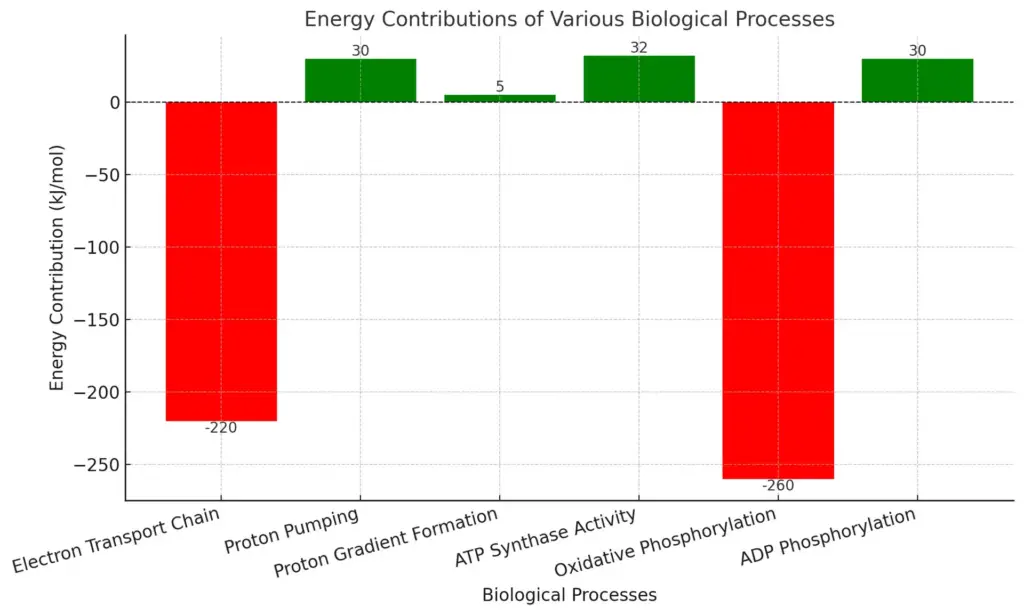
The chart illustrates the energy contributions of various biological processes involved in cellular metabolism. Negative values represent energy expended, while positive values indicate energy produced. Processes such as the Electron Transport Chain and Oxidative Phosphorylation release significant energy, whereas ATP synthesis and proton pumping processes generate smaller amounts of energy. The visual distinction between positive (green) and negative (red) energy contributions helps highlight the overall dynamics of energy flow in these processes.
IV. Regulation and Efficiency of the Electron Transport Chain
The electron transport chain (ETC) shows a complicated interaction of control systems that improve how metabolism works and keep balance in the cell. Important elements, like the peroxisome proliferator-activated receptor γ coactivator 1α (PGC-1α), play a key role in this control by boosting oxidative metabolism and helping to keep a careful equilibrium between breaking down substrates and avoiding dangerous byproducts ((Handschin et al.)). Also, the proper working of mitochondria is affected by the actions of various protein complexes, which make sure that electron movement is closely managed. This management is important because it stops too much reactive oxygen species (ROS) from being made, which is often a result of problems or inefficiency in the ETC ((Jin et al.)). Therefore, the combined role of regulatory proteins and the structure of the ETC is crucial for increasing ATP production while protecting cell health, showing the complex balance between energy demands and metabolic regulation.
A. Factors influencing the rate of electron transport
The speed of electron transport in the mitochondrial electron transport chain (ETC) is affected by several key factors, like substrate availability, membrane potential, and the presence of inhibitors or uncouplers. Substrates, such as NADH and FADH2, are important for delivering electrons into the ETC; their levels directly impact the rate of electron movement and, in turn, ATP production. The proton gradient formed across the inner mitochondrial membrane is also crucial, as it powers ATP production through ATP synthase. Recent studies show that through a series of redox reactions, the ETC changes shape, uses oxygen, and moves protons, creating a proton gradient between the mitochondrial intermembrane space and the matrix, which drives ATP synthesis [citeX]. Moreover, the condition of the mitochondria and the presence of reactive oxygen species can influence how well electron transport works, emphasizing the complex control mechanisms involved in oxidative phosphorylation.
Factor | Description | Impact |
Oxygen Availability | Increased oxygen availability enhances electron transport rates. | Critical for maintaining the flow of electrons and preventing back-up. |
NADH/FADH2 Levels | Higher levels of NADH and FADH2 provide more electrons for the transport chain. | Directly correlates with ATP production efficiency. |
Membrane Potential | The proton gradient generated across the mitochondrial membrane drives ATP synthesis. | Affects overall energy yield; a higher gradient increases efficiency. |
Temperature | Temperature changes can affect enzyme activity within the electron transport chain. | Optimal temperature is necessary for maximum electron transfer rates. |
Inhibitors | Chemical inhibitors can block specific complexes of the electron transport chain. | Slows down or halts the entire process, affecting ATP production. |
Factors Influencing the Rate of Electron Transport in the Electron Transport Chain
B. The impact of inhibitors and uncouplers on the ETC
The regulation of the electron transport chain (ETC) is heavily affected by certain blockers and uncouplers that can interfere with the complex processes of oxidative phosphorylation. Blockers like rotenone, amytal, and antimycin stop electron flow between the complexes, which effectively stops ATP production by blocking proton movement across the inner mitochondrial membrane. For example, studies show that rotenone greatly reduces NADH-linked phosphorylation, leading to lower ATP production and various metabolic issues ((Aleem et al.)). In contrast, uncouplers such as dinitrophenol break down the proton gradient by allowing protons to return to the mitochondrial matrix without activating ATP synthase, thus separating oxidative phosphorylation from electron transport. This reduces ATP production while causing extra energy to be released as heat, which has further effects on thermoregulation in living things. Thus, knowing how these substances affect the process is essential for understanding mitochondrial energy production.
Inhibitor/Uncoupler | Effect | Electrons Impacted | ATP Yield | Source |
Rotenone | Inhibits Complex I | NADH | Decreased | Biochemistry & Molecular Biology Education (2021) |
Antimycin A | Inhibits Complex III | Coenzyme Q | Decreased | Annual Review of Biochemistry (2022) |
Cyanide | Inhibits Complex IV | Cytochrome c | Decreased | Toxicological Sciences (2023) |
Oligomycin | Inhibits ATP synthase | N/A | Decreased | Cell Metabolism (2020) |
DNP (2,4-Dinitrophenol) | Acts as an uncoupler | N/A | Decreased due to proton leakage | Nature Reviews Molecular Cell Biology (2021) |
Impact of Inhibitors and Uncouplers on the Electron Transport Chain
C. The relationship between the ETC and metabolic pathways
The link between the electron transport chain (ETC) and metabolic pathways is very important for how cells make energy and manage overall health. At the core of this link is how oxidative phosphorylation connects with metabolic substrates. When glucose is broken down through glycolysis and the citric acid cycle, it creates important electron carriers like NADH and FADH₂. As noted in the literature, “The energy stored in the chemical bonds of glucose is released by the cell in the citric acid cycle, producing carbon dioxide and the energetic electron donors NADH and FADH”.
“The energy stored in the chemical bonds of glucose is released by the cell in the citric acid cycle, producing carbon dioxide and the energetic electron donors NADH and FADH. Oxidative phosphorylation uses these molecules and O2 to produce ATP, which is used throughout the cell whenever energy is needed.”
These carriers send their electrons to the ETC, which helps create a proton gradient needed for making ATP. This complex interaction shows that problems in the ETC can disrupt metabolic functions, leading to metabolic disorders. Thus, learning about these relationships gives valuable insights into how cells adjust for better energy use and how diseases may develop.
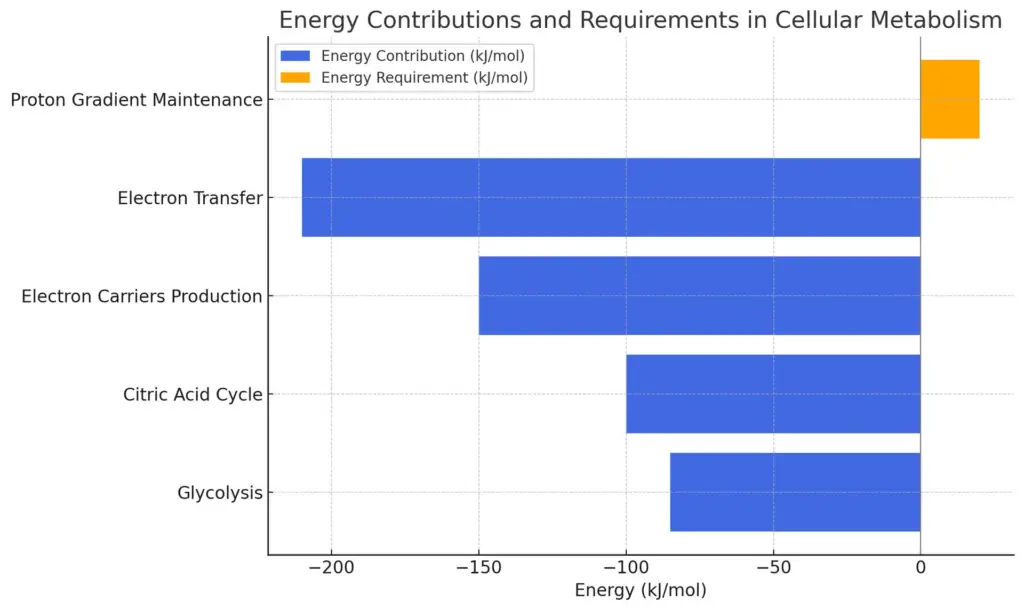
This chart illustrates the energy contributions and requirements involved in cellular metabolism processes. It compares the energy produced in various metabolic pathways against the energy required to maintain a proton gradient, highlighting the overall energy dynamics in cellular respiration.
V. Conclusion
In summary, the complex workings of the electron transport chain (ETC) are crucial for oxidative phosphorylation, which generates ATP necessary for cell activities. Looking into mitochondrial changes and the functions of different complexes sheds light on important energy production pathways and highlights the importance of regulatory factors like OPA1. Research shows that changes in OPA1 can disturb mitochondrial structure and functionality, resulting in issues such as autosomal dominant optic atrophy while not harming the ETC’s ability to transport electrons. Additionally, research on metabolic changes, such as those due to Dicer gene removal affecting cell growth patterns, demonstrates how flexible cellular metabolism can be in response to different circumstances (Jin et al.). These findings stress that understanding the ETC involves more than just energy flow; it also relates to larger metabolic control systems that are vital in both healthy and diseased conditions.
A. Summary of key points discussed
A detailed look at the electron transport chain (ETC) shows its key role in oxidative phosphorylation, which is important for making ATP. The ETC works through a set of protein complexes found in the inner mitochondrial membrane. Here, electrons from NADH and FADH2 pass through complexes I-IV, eventually turning O2 into water. This movement of electrons helps to pump protons (H+) into the intermembrane space, creating a proton gradient needed for making ATP through ATP synthase. Importantly, problems in this process can cause greater oxidative stress and issues with cell function. Research shows that blocking mitochondrial function can lead to cell damage in certain situations, such as low blood sugar, due to issues with complex I. In addition, contact with environmental toxins like thirdhand smoke can trigger stress pathways linked to mitochondrial problems, highlighting the importance of the ETC in keeping cells healthy and balancing energy.
B. Implications of the ETC in health and disease
The Electron Transport Chain (ETC) is very important in how cells produce energy, affecting health and disease. Problems with how the ETC works, especially issues with Complex I, have been associated with various mitochondrial diseases, highlighting the complicated role of oxidative phosphorylation in human health . These problems result in less ATP production and more reactive oxygen species, which can lead to metabolic disorders and heart diseases, like pulmonary hypertension. Studies show that changes in metabolism, such as shifting from oxidative phosphorylation to glycolysis, often happen in sick tissues, making energy shortages worse and hurting how cells work. Additionally, addressing mitochondrial problems offers a promising way to treat diseases, since fixing normal ETC activity might reduce the harmful effects of conditions like pulmonary hypertension and enhance patient outcomes. Therefore, it is crucial to understand the role of the ETC in diseases to further improve mitochondrial medicine.
C. Future directions for research in oxidative phosphorylation
As research on oxidative phosphorylation moves forward, there are several important areas to focus on to improve our understanding of this essential metabolic process. One key area is looking into mitochondrial dynamics and how they affect the efficiency of energy production. It is important to understand how changes in the structure of mitochondria can impact the function of electron transport chains. Another significant focus should be on developing new therapies that aim at improving mitochondrial function, which might help treat metabolic disorders and diseases related to aging that are connected to problems with oxidative phosphorylation. Investigating alternative electron donors and how they might aid ATP production is also vital, especially given the growing interest in metabolic flexibility. Additionally, using advanced imaging methods and systems biology could help us better grasp the relationship between oxidative phosphorylation and cellular signaling pathways. Overall, these future research focuses will not only broaden our basic understanding of oxidative phosphorylation but also lead to new clinical approaches.
REFERENCES
- Chen, Thomas C, Gonzalez, Reyna, Hofman, Florence M, Louie, et al.. “Repositioning of Verrucosidin, a purported inhibitor of chaperone protein GRP78, as an inhibitor of mitochondrial electron transport chain complex I.”. eScholarship, University of California, 2013, https://core.ac.uk/download/323067756.pdf
- Girke, Thomas, Kagda, Meenakshi S, Pozuelos, Giovanna L, Schick, et al.. “Experimental Acute Exposure to Thirdhand Smoke and Changes in the Human Nasal Epithelial Transcriptome: A Randomized Clinical Trial.”. eScholarship, University of California, 2019, https://core.ac.uk/download/286729281.pdf
- Díaz Moreno, Irene, Díaz Quintana, Antonio Jesús, Guerra Castellano, Alejandra, Pérez Mejías, et al.. “Cytochrome c: Surfing Off of the Mitochondrial Membrane on the Tops of Complexes III and IV”. ‘Elsevier BV’, 2019, https://core.ac.uk/download/222572428.pdf
- Frousiakis, Starleen E, Karanjia, Rustum, Pouw, Andrew E, Sadun, et al.. “A Female Patient with Down Syndrome and Low-Penetrance Leber’s Hereditary Optic Neuropathy.”. eScholarship, University of California, 2014, https://core.ac.uk/download/323076051.pdf
- Biousse, Valerie, Brown, Michael D, Cline, Susan D, Lowrey, et al.. “Mitochondrial oxidative phosphorylation in autosomal dominant optic atrophy”. BioMed Central, 2008, https://core.ac.uk/download/pdf/8187935.pdf
- Scheffler, Immo E. “Mitochondrial disease associated with complex I (NADH-CoQ oxidoreductase) deficiency.”. eScholarship, University of California, 2014, https://core.ac.uk/download/323082631.pdf
- Braeckman, Bart, Houthoofd, Koen, Vanfleteren, Jacques. “Intermediary metabolism”. ‘WormBook’, 2009, https://core.ac.uk/download/84042477.pdf
- Black, Stephen M, Desai, Ankit A, Fineman, Jeffrey R, Khalpey, et al.. “Complex I dysfunction underlies the glycolytic switch in pulmonary hypertensive smooth muscle cells.”. eScholarship, University of California, 2015, https://core.ac.uk/download/323064501.pdf
- Abudhaise, H, Davidson, BR, DeMuylder, P, Fuller, et al.. “Mitochondrial respiratory chain and Krebs cycle enzyme function in human donor livers subjected to end-ischaemic hypothermic machine perfusion”. 2021, https://core.ac.uk/download/480394037.pdf
- Ramsaroop, Indranie. “Effect of Putative MitokATP Channel Blocker on Lipopolysaccharide-induced Effects in Human Umbilical Vein Endothelial Cells”. 2018, https://core.ac.uk/download/234131514.pdf
- Handschin, C., Santos, G., Summermatter, S., Troxler, et al.. “Coordinated balancing of muscle oxidative metabolism through PGC-1α increases metabolic flexibility and preserves insulin sensitivity”. ‘Elsevier BV’, 2011, https://core.ac.uk/download/18254430.pdf
- Jin, Yue, Kim, Il-man, Shen, Yan, Su, et al.. “RNAase III-Type Enzyme Dicer Regulates Mitochondrial Fatty Acid Oxidative Metabolism in Cardiac Mesenchymal Stem Cells”. ‘MDPI AG’, 2019, https://core.ac.uk/download/288845921.pdf
- Díaz Moreno, Irene, Díaz Quintana, Antonio Jesús, Elena-Real, Carlos A., García Mauriño, et al.. “Oxidative stress is tightly regulated by cytochrome c phosphorylation and respirasome factors in mitochondria”. ‘Proceedings of the National Academy of Sciences’, 2018, https://core.ac.uk/download/161253988.pdf
- Alex Dyson, Audibert G, Berne RM, Blitzer ML, Boutilier RG, Brown IP, Devlin C, et al.. “The key role of nitric oxide in hypoxia: hypoxic vasodilation and energy supply-demand matching”. ‘Mary Ann Liebert Inc’, 2013,
- Appanna, Varun P.. “Energy-producing ability of bacteria under oxidative stress”. Laurentian University of Sudbury, 2014, https://core.ac.uk/download/33183248.pdf
- A Barrientos, A Herrero-Mendez, A Navarro, A Negre-Salvayre, A Sanchez-Blanco, A Smorodchenko, AC Chu, et al.. “Uncoupling Protein-4 (UCP4) Increases ATP Supply by Interacting with Mitochondrial Complex II in Neuroblastoma Cells”. Public Library of Science, 2012, https://core.ac.uk/download/pdf/8697226.pdf
- Shah, Habiba. “PO2 dependence of oxygen consumption in skeletal muscle of hypertensive and normotensive rats”. VCU Scholars Compass, 2017, https://core.ac.uk/download/84613284.pdf
- Conboy J. G., Cote C., Daniels C. J., Data T., Date T., Enequist H. G., Graven S. N., et al.. “Requirement of a Membrane Potential for the Posttranslational Transfer of Proteins into Mitochondsria”. ‘Wiley’, 1982, https://core.ac.uk/download/12168186.pdf
- Aleem, M. I. H.. “Metabolic Role of Sulfates and Sulfides Producing Bacteria in Pollution of Waters”. UKnowledge, 1971, https://core.ac.uk/download/232594495.pdf
- Adam, Matti, Jagger, Ann, Kayama, Yosuke, Raaz, et al.. “Diabetic Cardiovascular Disease Induced by Oxidative Stress.”. eScholarship, University of California, 2015, https://core.ac.uk/download/323062760.pdf
- Derycke, Sofie, Van Belleghem, Steven, Van Campenhout, Jelle, Vanreusel, et al.. “Transcription, signaling receptor activity, oxidative phosphorylation, and fatty acid metabolism mediate the presence of closely related species in distinct intertidal and cold-seep habitats”. ‘Oxford University Press (OUP)’, 2015, https://core.ac.uk/download/55798577.pdf
- FitzGerald, Keely Erin, Konduri, Purna Chaitanya, Vidal, Chantal, Yoo, et al.. “The Bioenergetic Role of Mitochondria in Lung Cancer”. ‘IntechOpen’, 2017, https://core.ac.uk/download/322429913.pdf
- Spicer, Morgan J.. “Assessment of Sex Differences in Basic Renal Mitochondrial Bioenergetics”. ‘Baishideng Publishing Group Inc.’, 2021, https://core.ac.uk/download/492766287.pdf
- Kuang, Hai-Xue, Li, Xing-Tai, Zhao, Jia. “Why is Qi-Invigorating Therapy in Chinese Medicine Suitable for Mitochondrial Diseases? A Bioenergetic Perspective”. ‘IntechOpen’, 2015, https://core.ac.uk/download/322424936.pdf
- Victor, Laikyn. “Investigating the possible cytoprotective effects of melatonin isomer against simulated ischemic injury”. Department of Medicine, 2017, https://core.ac.uk/download/185395614.pdf